|
|
|
ABSTRACT |
The primary objective of this study was to assess the efficacy of measuring both aerobic and anaerobic power in a 60-second, maximal effort test. It was hypothesized that oxygen consumption increases rapidly during maximal effort and maximal oxygen consumption (VO2 max) may be reached in one minute. Fifteen United States Cycling Federation competitive cyclists performed the following tests: 1) practice 60-second maximal exertion test; 2) standard incremental workload VO2 max test; 3) Wingate anaerobic power test (WAT); 4) VO2 measured during 60-second maximal exertion test (60-SEC); and 5) VO2 measured during 75-second maximal exertion test (75-SEC). All tests were performed on an electrically-braked cycle ergometer. Hydrostatic weighing was performed to determine percent body fat. Peak oxygen consumption values for the 60-SEC (53.4 ml·kg-1·min-1, 92% VO2 max), and 75-SEC (52.6 ml·kg-1·min-1, 91% VO2 max) tests were significantly lower than VO2 max (58.1 ml·kg-1·min-1). During the 75-SEC test, there was no significant difference in percentage VO2max from 30 seconds to 75 seconds, demonstrating a plateau effect. There were no significant differences in peak power or relative peak power between the Wingate, 60-SEC, and 75 SEC tests while, as expected, mean power, relative mean power, and fatigue index were significantly different between these tests. Power measures were highly correlated among all three tests. It was concluded that VO2 max was not attained during either the 60-SEC nor 75-SEC tests. Furthermore, high correlations in power output for WAT, 60-SEC, and 75-SEC precludes the necessity for anaerobic tests longer than the 30-second WAT. |
Key words:
Maximal oxygen consumption, Wingate
|
Key
Points
|
Measurement of maximal oxygen consumption (VO2 max) has long been accepted as the “gold standard” in the assessment of cardio-respiratory fitness. It has been shown to be inversely related (especially in heterogonous competitors) to performance time in endurance cycling events (Craig et al., 1993; Hopkins and McKenzie, 1994). It has also been demonstrated to be associated with recovery time in intermittent events of high intensity, attenuating the decline in performance due to fatigue (DePampero and Margaria, 1968). Maximal oxygen consumption has traditionally been measured utilizing an incremental, continuous or discontinuous protocol performed to volitional fatigue. It is generally accepted that a plateau in oxygen consumption with increasing exercise intensity indicates attainment of true VO2 max, although this plateau is not always observed. Recent research has attempted to determine if VO2 max can be attained utilizing a supramaximal workload for a prescribed length of time in which the subject fatigues and work output decreases. While attainment of VO2 max in 60 seconds has been reported (Serresse et al., 1988), others have found a rapid increase in oxygen consumption, but failure to attain VO2 max values attained during an incremental exercise test to exhaustion (Gastin and Lawson, 1994a). A variety of test modes have been utilized to measure anaerobic capacity, including running (Medbo and Sejerstad, 1985; Scott et al., 1991; Olesen et al., 1994), stair climbing, and cycling (Serresse et al., 1988; Withers et al., 1993; Gastin and Lawson, 1994a; 1994b; Craig et al., 1995). However, the test most frequently cited as the standard in assessment of anaerobic capacity is the 30-second Wingate Anaerobic Power Test (WAT) (Vandewalle et al., 1985; Patton and Duggan, 1987; Bar-Or et al., 1988). The Wingate test has proven to be highly reliable (Patton et al., 1985; Bar-Or et al., 1988) and to correlate well with running tests of anaerobic power (Patton et al., 1985; Bar-Or et al., 1988; Scott et al., 1991). Testing of anaerobic power utilizing cycle ergometry has the advantage of 1) continuous power assessment throughout the test; 2) power adjustment based on fatigue as the test progresses (power output decreases as cadence decreases at a fixed torque) and 3) gas analysis measurement in a stationary subject. Recently, several investigators have proposed that the duration of an anaerobic capacity test should be longer than 30 seconds because the maximal accumulated oxygen deficit continues to increase after 30 seconds (Hill and Scarborough, 1986; Medbo and Tabata, 1989; Withers et al., 1991; Gastin et al., 1994a; 1994b; Weber and Schneider, 2001). This deficit is defined as the difference between oxygen consumption predicted from a linear extrapolation from submaximal to supramaximal workloads and the actual measured oxygen consumption (Medbo et al., 1988). While several investigators have supported the validity of using this measure in the assessment of anaerobic capacity (Scott et al., 1991; Medbo and Tabata, 1993; Gastin and Lawson, 1994a; 1994b), others have refuted it (Withers et al., 1993; Green et al., 1996; Bangsbo, 1998). This is due to either a non-linear increase in O2 consumption above the anaerobic threshold (Bangsbo, 1998) or the failure of this technique to distinguish endurance-trained athletes from strength-trained athletes (Withers et al., 1993). This latter point has been refuted by Scott et al., (1991), who found a significant difference in maximal accumulated oxygen debt between middle-distance and long-distance runners. The ideal duration of a maximal effort test to assess anaerobic capacity via the maximal accumulated oxygen deficit (MAOD) may range from 1 to 2 minutes, although 80% of the maximal accumulated oxygen deficit can be attained in the first 30 seconds of a maximal effort test (Gastin and Lawson, 1994b). The primary objective of this study was to assess the efficacy of a one-minute maximal effort test (60 SEC) for assessing both aerobic and anaerobic power. A 75 second maximal effort test (75-SEC) was also included in the research protocol in the event that the 60-SEC test was of insufficient duration to attain VO2 max. While assessment of maximal accumulated oxygen deficit and the determination of optimal test duration for measuring anaerobic capacity were beyond the scope of this study, previous research supports the use of 60-second, maximal effort test, rather than a 30-second test for assessment of anaerobic capacity (Scott et al., 1991; Withers et al., 1991; Medbo and Tabata, 1993; Gastin and Lawson, 1994a; 1994b; Weber and Schneider, 2001). Given that previous research has supported a very rapid increase in oxygen consumption, perhaps attaining VO2 max in 60 seconds (Serresse et al., 1988; Gastin and Lawson, 1994a), and that anaerobic capacity may best be measured by a 60-second rather than 30-second, maximal effort test (Hill et al., 1986, Medbo and Tabata, 1989; Withers et al., 1991; Gastin and Lawson, 1994b; Weber and Schneider, 2001), this study was designed to determine if both aerobic and anaerobic capacity could be measured with a single, maximal effort test of 60 seconds. The results of this study may, in effect, eliminate the necessity of subjects performing 2 independent maximal effort tests, thereby reducing both costs and the necessity of two exhaustive, fatiguing exercise tests.
Study ParticipantsThe physical characteristics of the subjects are listed in Table 1. All participants (n = 15; n = 13 men and N= 2 women; mean ± SD for age = 33.2 ± 8.6 years) were United States Cycling Federation (USCF) cyclists who were in the midst of their competitive season at the time of data collection. All cyclists competed in endurance events ranging form 16 minutes to ultra-endurance competition. Of the 15 cyclists, two were category V, seven were category IV, four were category III, and two were category II, according to the United States Cycling Federation. In this rating system, cyclists start at category V and move to categories IV and III based on race results, number of races participated in, or a combination of both. Cyclists move from category III to categories II and I based on race results only. All cyclists were racing competitively at the time of the study, participating in two to twelve races per month which included time trials, criteriums and road races. Due to the small number of female participants, data were analyzed for total subjects only. Approval to conduct this research was obtained from the Institutional Review Board (IRB) of the University of St. Thomas prior to data collection.
Study ProtocolEach participant made three visits to the University of St. Thomas Human Performance Laboratory. During visit one, subjects completed a brief training and racing history form, read and signed the consent form, and performed the 60-second maximal effort test to familiarize the subject to the test (FAM). The purpose of FAM was to negate any possible learning effects associated with performance of maximal effort tests (Martin et al., 2000). Procedures for this test were identical to the 60-SEC test described below. The second visit included an incremental exercise test to measure VO2 max, the Wingate Anaerobic Test to assess anaerobic capacity, and underwater weighing for the measurement of fat mass, lean body mass, and percent body fat. The protocol used to assess VO2 max consisted of one-minute, 25-watt increments in power output to exhaustion, beginning at 25 watts. During visit three, participants completed both the 60-SEC and 75-SEC tests in random order. All maximal exertion tests were separated by a one-hour rest period. For each participant, tests were completed at the same time of day and within a one-week period.
Maximal Exertion TestsTests were performed on an electrically-braked cycle ergometer (Lode BV, Groninger, The Netherlands). Subjects brought their own pedals and cycling shoes and adjusted seat and handlebars to their specifications. Gas analysis was performed with the Medical Graphics CPX-D Metabolic Measurement System (Medical Graphic Corp., St. Paul, Minnesota), which was calibrated for volume, room temperature and pressure, and gas concentration (12% O2, 5% CO2) prior to each test. Breath-by-breath analysis with 30-second averaging was done for the incremental VO2 max test. For both the 60-SEC and 75-SEC tests, breath-by-breath analysis and 3-second averaging was also performed. The incremental VO2 max test began at 25 watts and increased 25 watts per minute to volitional fatigue. All subjects met at least two of the following three criteria for attainment of VO2 max: 1) attainment of 90% age-predicted maximum heart rate; 2) plateauing of oxygen consumption (<150 ml·min-1 difference in oxygen consumption for the final two stages; and 3) respiratory quotient (RQ) greater than 1.1. Maximal oxygen consumption was determined to be the highest VO2 attained during a 30-second interval. Determination of optimal resistance for assessment of both peak power and mean power for the 60-SEC and the 75-SEC tests was based on previous research (Gastin et al., 1991) and were set at .075 g·kg-1 for men and .065 g·kg-1 for women, respectively. Mean values for resistance, for the 60-SEC and 75-SEC tests were 58.5 and 57.8 Newton·meters (N·m), respectively (n = 15). Resistance for the Wingate Test was standardized according to software manufacturer recommend-ations (male torque factor = .8, female torque factor = .77) and resulted in a mean value of 60.6 N·m. Differences in torque for the three tests were relatively small but significant (p < .05). Heart rates (Polar Electro, Woodbury, New York) were measured the last 5 seconds of each stage and at volitional fatigue for the incremental exercise test and at the completion of the 60-SEC and 75-SEC tests.
Body CompositionBody composition was determined from hydrostatic weighing. The method for hydrostatic weighing has been previously described (Foss et al., 1998). Residual volumes for both men and women were predicted from sex-specific formulas according to methods previously determined by Weidman et al. (1987). Percent body fat was predicted from body density by the Siri equation (Siri, 1956). Fat mass was calculated by multiplying percent body fat by body weight. Lean body mass was determined by subtracting FM from body weight.
Data AnalysisThe physical characteristics of the subjects and their performance data are presented as means (SD). Differences in oxygen consumption values between the incremental VO2 max, 60-SEC and 75-SEC tests were evaluated using Analysis of Variance with repeated measures (Statistix Analytical Software, Tallahassee, Florida). Differences between the Wingate, 60-SEC and 75-SEC tests for the various power output measures assessed were evaluated using this same Statistix analytical software program. When significant differences were found for Analysis of Variance, post-hoc t-tests were performed using a Bonferroni correction factor. The relationships between power output values attained during the VO2 max, 30-sec. Wingate test, 60- and 75-SEC tests were evaluated using the Pearson Product Moment Correlation Coefficient. Statistical significance was accepted at the p < .05 level.
Aerobic PowerExertional measures for the incremental VO2 max, 60-SEC, and 75-SEC tests are shown in Table 2. Mean VO2 max for the incremental test (58.1 ml·kg-1 ·min-1) was significantly higher than mean VO2 max values obtained during the 60-SEC (53.4 ml·kg-1 ·min-1, p < .0001, t=6.44) and 75-SEC (52.6 ml·kg-1 ·min-1, p < .0001, t=5.53) tests. There was no significant difference between the mean oxygen consumption attained during the 60- and 75-SEC tests (p = .407, t=0.85). While there was no significant difference in oxygen consumption values for the 75-SEC test from 30 seconds (87.0%) to 75 seconds (91.0%), the data indicate a slight increase in oxygen consumption values during this time period (Table 3).
Anaerobic PowerMean peak power (PP) (p = 0.01), but not mean of the mean power (MP) (p = 0.53) was significantly greater for 60-SEC than FAM, supporting the presence of a learning curve in producing maximal power and justifying the use of a familiarization session prior to actual data collection (Martin et al., 2000.). Several indices of power output determined from the Wingate, 60-SEC, and 75-SEC tests are shown in Table 4. As expected, mean power, relative mean power, and minimum power were all significantly greater for the Wingate test compared to the 60-SEC and 75-SEC tests. Conversely, the fatigue index was significantly lower for the Wingate compared to the 60-SEC and 75-SEC tests. No significant differences were observed for peak power between the three tests. For each index of power, there was a high degree of co-linearity between the Wingate, 60-SEC, and 75-SEC tests (Table 5). The relationship between aerobic power (VO2 max) and the various indices of power output assessed during the Wingate, 60-SEC, and 75-SEC tests were small and not statistically significant, with the exceptions of relative mean power for 60-SEC (r = .52) and 75-SEC (r = .53). While these correlation coefficients were significant, they are relatively low, and probably of little practical value.
The major finding of this study was that 60 and 75 seconds of maximal effort cycling achieved only 92% and 91%, respectively, of VO2 max attained during traditional, incremental VO2 max testing. It should be noted that while 91.0% VO2 max was attained in 75 seconds, 87% VO2 max was attained in just 30 seconds. This demonstrates an initial rapid increase in VO2, which quickly plateaus with increasing duration. These results are: 1) nearly identical to those obtained by some investigators (Gastin et al., 1991, Withers et al., 1991), 2) somewhat higher than those obtained by Withers et al. (1993) and 3) somewhat lower than those obtained by Serresse et al. (1988), who reported the attainment of VO2 max in 60 seconds in trained cross-country skiers, biathletes, and speed skaters using cycle ergometry. Others have contended that at least 2 minutes of supramaximal exercise are needed to attain VO2 max (Astrand and Saltin, 1961). There are several potential reasons, which may explain the failure to achieve VO2 max in 60 or 75 seconds observed in the present study. The following discussion is speculative in nature and offers several possible explanations for these findings. Rapid accumulation of hydrogen ions in muscle, resulting in acidosis, may have precluded the attainment of VO2 max (Hermanson, 1969). The highest recorded blood lactate levels (32 mM·L-1) have been found in well-trained athletes during recovery from competitive events lasting approximately one minute (Osnes and Hermansen, 1972), which is comparable to the 60-SEC and 75-SEC tests used in the present study. Lower cardiac outputs may have been achieved during the 60-SEC and 75-SEC tests, contributing to the failure to achieve VO2 max. While cardiac output was not assessed in the present study, it would be predicted to be lower due to the lower maximal heart rates achieved during the 60-SEC and 75-SEC tests compared to the incremental VO2 max test (Table 2). It should be noted that the percentage VO2 max attained during the 60-SEC (92%) and 75-SEC (91.0%) tests were similar to the percentage maximal heart rates achieved during these tests (96% and 94%, respectively, Table 2). Arterial oxygen desaturation may have occurred due to respiratory fatigue from prolonged hyperventilation, which would preclude the attainment of VO2 max. Hyperventilation has been shown to increase the ratio of dead space to tidal volume (Wasserman et al., 1986). During the 75-SEC test, with the exception of a significant decrease in ventilation from 60 seconds to 75 seconds (164.5 vs. 159.5 Liters per minute), no significant differences in tidal volume, respiratory rate, and ventilation were observed from 45 seconds to 75 seconds, indicating a plateau in these variables for the last 30 seconds of the test. A significant drop in ventilation from 60 to 75 seconds may indicate respiratory fatigue. While fatigue of the respiratory muscles has been controversial, those studies examining respiratory fatigue during incremental exercise have concluded that "the high levels of ventilation observed during maximal exercise are not maintained for sufficient time to result in mechanical failure"(Younes and Kivinen, 1984). Maximal ventilation was maintained considerably longer in this study, as determined by attainment of maximal ventilation at 30 seconds of the 75-SEC test. Ventilations at 30, 45, 60, and 75 seconds of the 75-SEC test were not significantly different from the maximum ventilation achieved during the VO2 max test. However, tidal volumes were significantly less (p < .05) and respiratory rates were significantly greater (p < .05) for all of these intervals during the 75-SEC test, compared to maximal values achieved during the VO2 max test. This hyperventilation during the 75-SEC test would explain the rapid and significant (p < .05) increase seen in end-tidal oxygen fraction (15.5%, 17.1%, 16.7%, and 16.5% at 0, 30,60, and 75 seconds, respectively). These responses may reflect gas exchange conditions that possibly resulted in a degree of oxygen-hemoglobin desaturation and a decreased delivery of oxygen to working muscle. While hemoglobin desaturation has been reported to occur in a minority of well-trained athletes (Dempsey et al., 1984, Turcott et al., 1997, Wetter et al., 2001), these studies used either steady state exercise at submaximal intensity, or incremental exercise to exhaustion, in contrast to the supramaximal intensity of the present study. The reasons for the discrepancies between the results of the present study and those of Serresse et al. (1988), who reported the attainment of VO2 max in 60 seconds, remain uncertain. Differing results may be due to differences in testing protocol. For instance, Serresse states that "differences may be explained…. by pacing during the first 20-30 sec of the 90-sec test." This is in contrast to the present study, which incorporated a maximal effort applied immediately at the beginning of the 60-SEC and 75-SEC test. Pacing may have allowed for a gradual increase in VO2 that would simulate an incremental protocol. The negative correlations obtained for absolute peak power and absolute mean power, when compared to VO2 max, is not surprising, given that the subjects in this study were endurance-trained cyclists. However, these negative correlations become slightly positive when expressed as relative peak power and relative mean power. This may best be explained by the fact that body weight is included in both variables (VO2 max expressed as ml·kg-1 ·min-1 and power as watts·kg-1). Correlation coefficients of .629 and .764 were obtained for comparisons of weight to peak power and weight to mean power, respectively in this group of subjects.
From the results of this study, it is concluded that aerobic and anaerobic power cannot be measured in a single 60-second supramaximal effort test. While it might be speculated that 60 seconds is of such short duration that it is not possible to go from a submaximal, low-level oxygen consumption to the high values attained by well-trained endurance athletes in this time period, the fact that 87% of VO2 max can be attained in 30 seconds would indicate that other factors are operative besides time that accounts for this failure to attain VO2 max. Future studies should be directed to ascertain what physiological mechanisms are operative in prevention of attainment of true VO2 max.
|
AUTHOR BIOGRAPHY |
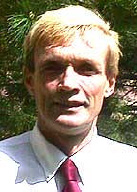 |
Daniel G. Carey |
Employment: Ass. Prof. in the Department of Health and Human Performance at the University of St. Thomas in St. Paul, Minnesota, USA. |
Degree: PhD |
Research interests: Anaerobic threshold and body composition assessment. |
E-mail: dgcarey@stthomas.edu |
|
 |
Mark T. Richardson |
Employment: Ass. Prof. in the Department of Human Performance Studies at the University of Alabama in Tuscaloosa, Alabama, USA. |
Degree: Ph.D. |
Research interests: Physical activity and cardiovascular epidemiology |
E-mail: mrichard@bamaed.ua.edu |
|
|
|
REFERENCES |
 Astrand P. O., Saltin B. (1961) Oxygen uptake during the first minutes of heavy muscular exercise. Journal of Applied Physiology 16, 971-976.
|
 Bangsbo J. (1998) Quantification of anaerobic energy production during intense exercise. Medicine and Science in Sports and Exercise 30, 47-52.
|
 Bar-Or O., Dotan R., Inbar O. (1988) A 30-sec all-out ergometric test: Its reliability and validity for anaerobic capacity. Israel Journal of Medicine and Science 13, 326-327.
|
 Craig N., Norton K., Conyers R., Woolford S., Bourdon P., Stone T., Walsh C. (1995) Influence of test duration and even specificity on maximal accumulated oxygen deficit of high performance track cyclists. International Journal of Sports Medicine 16, 534-540.
|
 Craig N., Pyke F., Norton K. (1989) Specificity of test duration when assessing the anaerobic lactacid capacity of high-performance track cyclists. International Journal of Sports Medicine 10, 237-242.
|
 Craig N., Pyke F., Norton K., Bourdon P., Woodford S., Stanef T., Squires B., Oldis T., Conyers R., Walsh C. B. (1993) Aerobic and anaerobic indices contributing to track endurance cycling performance. European Journal of Applied Physiology and Occupational Physiology 67, 150-158.
|
 Dempsey J., Hanson P., Henderson K. (1984) Exercise-induced arterial hypoxemia in healthy human subjects at sea level. Journal of Physiology 355, 161-175.
|
 DePampero P., Margaria R. (1968) Relationship between O consumption, high energy phosphates, and the kinetics of the O debt in exercise. Pfluger Archive 304, 11-19.
|
 Foss M., Keteyian S. (1998) Exercise, body composition, and weight control. Physiological Basis for Exercise and Sport , 422-446.
|
 Gastin P., Lawson D. (1994a) Variable resistance all-out test to generate accumulated oxygen deficit and predict anaerobic capacity. European Journal of Applied Physiology 69, 331-336.
|
 Gastin P., Lawson D. (1994b) Influence of training status on maximal accumulated oxygen deficit during all-out cycle exercise. European Journal of Applied Physiology 69, 321-330.
|
 Gastin P., Lawson D., Hargreaves M., Carey M., Fairweather I. (1991) Variable resistance loadings in anaerobic power testing. International Journal of Sports Medicine 12, 513-518.
|
 Green S., Dawson B., Goodman C., Carey M. (1996) Anaerobic ATP production and accumulated O deficit in cyclists. Medicine and Science in Sports and Exercise 28, 315-321.
|
 Hermanson L (1969) Anaerobic energy release. Medicine and Science in Sports and Exercise 1, 32-38.
|
 Hill D., Smith J., Scarborough P. (1986) Optimal exercise duration for determination of anaerobic capacity in men and women. Medicine and Science in Sports and Exercise 22, S34-.
|
 Hopkins S., McKenzie D. (1994) The laboratory assessment of endurance performance in cyclists. Canadian Journal of Applied Physiology 19, 266-274.
|
 Martin J., Diedrich D., Coyle E. (2000) Time course of learning to produce maximum cycling power. International Journal of Sports Medicine 21, 485-487.
|
 Medbo J., Mohn A., Tabata I., Bahr R., Vaage O., Sejersted O. (1988) Anaerobic capacity determined by maximal accumulated O deficit. Journal of Applied Physiology 64, 50-60.
|
 Medbo J., Sejersted O. (1985) Acid-base and electrolyte balance after exhausting exercise in endurance-trained and sprint-trained subjects. Acta. Physiologica Scandinavica 125, 97-109.
|
 Medbo J., Tabata I. (1989) Relative importance of aerobic and anaerobic energy release during short-lasting exhausting bicycle exercise. Journal of Applied Physiology 67, 1881-1886.
|
 Medbo J., Tabata I. (1993) Anaerobic energy release in working muscle during 30 to 3 minute of exhausting bicycling. Journal of Applied hysiology 75, 1654-1660.
|
 Olesen H., Raabo E., Bangsbo J., Secher N. (1994) Maximal oxygen deficit of spring and middle distance runners. European Journal of Applied Physiology 69, 140-146.
|
 Osnes J., Hermansen L. (1972) Acid-base balance after maximal exercise of short duration. Journal of Applied Physiology 32, 59-63.
|
 Patton J., Duggan A. (1987) An evaluation of tests of anaerobic power. Aviation, Space and Environmental Medicine 58, 237-242.
|
 Patton J., Murphy M., Frederick F. (1985) Maximal power outputs during the Wingate Anaerobic test. International Journal of Sports Medicine 6, 82-85.
|
 Scott C., Roby F., Lohman T., Bunt J. (1991) The maximally accumulated oxygen deficit as an indicator of anaerobic capacity. Medicine and Science in Sports and Exercise 23, 618-624.
|
 Serresse O., Lortie G., Bouchard C., Boulay M. (1988) Estimation of the contribution of the various energy systems during maximal work of short duration. International Journal of Sports Medicine 9, 456-460.
|
 Siri W., Lawrence J.H., Tobias C.A. (1956) Advances in Biological and Medical Physics. Gross composition of the body. New York. Academic Press.
|
 Turcott R., Kiteala J., Marcotte J., Perrault H. (1997) Exercise-induced oxyhemoglobin desaturation and pulmonary diffusing capacity during high-intensity exercise. European Journal of Applied Physiology and Occupational Physiology 75, 425-430.
|
 Vandewalle H., Peres G., Heller J., Monod H. (1985) All out anaerobic capacity tests on cycle ergometers. European Journal of Applied Physiology 54, 222-229.
|
 Wasserman K., Hansen J., Sue D., Whipp B. (1986) Principles of exercise testing and interpretation. Philadelphia, Pa.. Lea and Febiger.
|
 Weber C., Schneider D. (2001) Reliability of MAOD measured at 110% and 120% of peak oxygen uptake for cycling. Medicine and Science in Sports and Exercise 33, 1056-1059.
|
 Weidman D., Tesch J., Castek J., Wilson P., Buckenmeyer P. (1987) A formula for the prediction of residual volume in males. Medicine and Science in Sports and Exercise 19, S31-.
|
 Wetter T., St. Croix C., Pegelow D., Sonnetti D., Dempsey J. (2001) Effects of exhaustive endurance exercise on pulmonary gas exchange and airway function in women. Journal of Applied Physiology 9, 847-858.
|
 Withers R., Sherman W., Clark D., Esselbach P., Nolan S., Mackay M., Brinkman M. (1991) Muscle metabolism during 30, 60, and 90s of maximal cycling on an air-braked ergometer. European Journal of Applied Physiology 63, 354-362.
|
 Withers R., Van Der Ploeg G., Finn J. (1993) Oxygen deficits incurred during 45, 60, 75, and 90s maximal cycling on an air-braked ergometer. European Journal of Applied Physiology 67, 185-191.
|
 Younes M., Kivinen G. (1984) Respiratory mechanics and breathing pattern during and following maximal exercise. Journal of Applied Physiology: Respiratory and Environmental exercise Physiology 57, 1773-1782.
|
|
|
|
|
|
|