|
|
|
ABSTRACT |
The purpose of the study was to investigate the influence of training with reduced breathing frequency (RBF) on tidal volume during incremental exercise where breathing frequency was restricted and on ventilatory response during exercise when breathing a 3% CO2 mixture. Twelve male participants were divided into two groups: experimental (Group E) and control (Group C). Both groups participated three cycle ergometry interval training sessions per week for six weeks. Group E performed it with RBF i.e. 10 breaths per minute and group C with spontaneous breathing. After training Group E showed a higher vital capacity (+8 ± 8%; p = 0.02) and lower ventilatory response during exercise when breathing a 3% CO2 mixture (-45 ± 27%; p = 0.03) compared with pre-training. These parameters were unchanged in Group C. Post-training peak power output with RBF (PPORBF) was increased in both groups. The improvement was greater in Group E (+42 ± 11%; p < 0.01) than in Group C (+11 ± 9%; p = 0.03). Tidal volume at PPORBF was higher post-training in Group E (+41 ± 19%; p = 0.01). The results of the present study indicate that RBF training during cycle ergometry exercise increased tidal volume during incremental exercise where breathing frequency was restricted and decreased ventilatory sensitivity during exercise when breathing a 3% CO2 mixture. |
Key words:
Interval training, reduced breathing, incremental exercise
|
Key
Points
- Training with a reduced breathing frequency during exercise decreased ventilator sensitivity to carbon dioxide. In addition, it increased minute ventilation during exercise with imposed reduced breathing frequency.
- Training with reduced breathing frequency could not be realized at higher intensity of exercise due to the additional stress caused by such a breathing pattern. Therefore the improvement in aerobic endurance (considering peak oxygen uptake) could not be expected after this kind of training.
|
Perhaps the best example in sport of where breathing frequency is naturally reduced is during front crawl swimming. During such exercise breathing (and specifically inspiration) must be coordinated with stroke mechanics and as a result tidal volume (VT) is increased to compensate for the reduced breathing frequency (RBF) (Dicker et al., 1980). Such a restricted breathing pattern during front crawl swimming limits minute ventilation (VE) and can lead to hypercapnia i.e. carbon dioxide retention (Cordain and Stager, 1988). The breathing pattern that swimmers adopt during front crawl largely depends on the swimming distance. For example, it is usual practice for swimmers to breathe every second stroke cycle during events of 200 metres or more, but to adopt a more restricted pattern during shorter front crawl distances (Maglischo, 2003). Consequently, it is not surprising that coaches have included RBF sets i.e. taking a breath every fourth, fifth, sixth or eighth stroke cycle during training: a practise which has been used in swimming since the 1970’s. Several studies have examined the influence of RBF on different physiological parameters in swimming since then. RBF during swimming reduced VE, increased the alveolar CO2 concentration (Cordain and Stager, 1988; Holmér and Gullstrand, 1980; Peyrebrune et al., 2003; Town and VanNess, 1990) and induced higher partial pressures of carbon dioxide in capillary blood (Kapus et al., 2002; 2003). Due to the technical limitations of measuring respiratory and blood parameters during swimming, the impact of RBF on physiological parameters has been investigated during cycle ergometry test (Kapus et al., 2009; 2010a; 2010b; Sharp et al., 1991; Yamamoto et al., 1987; 1988) and treadmill running (Matheson and McKenzie, 1988): here breathing frequency can be modified and respiratory and blood parameters measured with greater ease. These studies confirmed the presence of marked hypercapnia as a result of RBF during exercise. In addition, these studies also examined hypoxemia by measuring capillary blood Po2 and oxygen saturation during exercise with RBF (Kapus et al., 2009; 2010a; 2010b; Matheson and McKenzie, 1988; Sharp et al., 1991; Yamamoto et al., 1987). All of the above studies investigated the acute effects of RBF. To our knowledge only one study has examined the influence of RBF training on exercise performance and did so in swimming. After four weeks of RBF training (breathing every fourth stroke cycle during front crawl) swimmers reduced their breathing frequency from 32 ± 5 breaths per minute to 25 ± 7 breaths per minute during a maximal 200 meter front crawl swim (Kapus et al., 2005). Although the mechanisms responsible for this training adaptation are not clear, it is possible that RBF training increased tidal volume and did so sufficiently to the extent that VE was increased. Given that RBF creates a hypercapnic training stimulus (Dicker et al., 1980; Peyrebrune et al., 2003) it is possible that the adaptation to hypercapnia could be the result of RBF training. Consequently the purpose of this study was twofold: firstly, to investigate the influence of RBF training on VT during incremental exercise where breathing frequency is restricted. Secondly, to investigate the effect of RBF training on the ventilatory response during exercise when breathing a 3% CO2 mixture, which is thought to be an indicator of ventilatory sensitivity to hypercapnia (Florio et al., 1979). Considering suggestions from previous studies, we hypothesise that RBF training will increase VT during incremental exercise where breathing frequency is restricted and decrease ventilatory sensitivity during exercise when breathing a 3% CO2 mixture.
Participants Twelve healthy male participants volunteered to participate in this study. As students of the Faculty of Sport, they were active but none were currently participating in a regular training programme. During the study period, all participants stopped their usual physical activity (recreational) and only exercised during the training sessions as part of the experiment. Descriptive measures of participants and training groups are presented in Table 1. None of the participants were smokers and all were free from respiratory diseases. They were fully informed of the purpose and possible risks of the study before giving their written consent to participate. The study was approved by the National Medical Ethics Committee of Slovenia.
Testing protocolAll testing and training was performed on an electromagnetically braked cycle ergometer (Ergometrics 900, Ergoline, Windhagen, Germany) with a pedal cadence of 60 rpm. Participants completed the following exercise tests in the same order pre- and post-training: 1) an incremental test to obtain peak power output with spontaneous breathing (SB) (PPOSB) and peak oxygen uptake (VO2 peak); 2) an incremental test to obtain peak power output with RBF (PPORBF); and 3) a constant load test with SB to determine the ventilatory response during exercise when breathing a 3% CO2 mixture (ventilatory sensitivity). At pre-training testing, two additional tests were performed: 1) a constant load test with SB at PPOSB; 2) a constant load test with RBF at PPORBF. The data obtained from these tests were used for determining the training interval sets only. Each exercise test was performed on a different day. All testing took place under controlled environmental laboratory conditions (21°C, 40-60% RH, 970-980 mbar) and at the same time of day. Participants were asked to maintain their usual eating habits and to avoid consuming food 2 hours before testing. Post-training testing began 2 days after the last training session. RBF was defined as 10 breaths per minute and was regulated by a breathing metronome. The breathing metronome was composed of a gas service solenoid valve (24 VDC, Jakäa, Ljubljana, Slovenia) and a semaphore with red and green lights. Both were controlled by micro-automation (Logo DC 12/24V, Siemens, Munich, Germany). The participants were instructed to exhale and inhale during a two second period of open solenoid valve (the green semaphore light was switched on) and to hold their breath, using almost all lung capacity (holding breath near total lung capacity), for four seconds when the solenoid valve was closed (the red semaphore light was switched on). Prior to testing and training, the participants were familiarized with cycling on the cycle ergometer and breathing in time with the metronome. Incremental exercise test: The participants initially performed an incremental exercise test with SB to obtain VO2 peak and PPOSB. The test began at an intensity of 30 W and increased by 30 W every two minutes until volitional exhaustion. VO2 peak was defined as the highest oxygen uptake averaged over a 60 second interval. On the next testing day, the participants performed an incremental exercise test with RBF to obtain PPORBF. With the exception of breathing pattern, the exercise protocols of the two tests were identical. In both incremental exercise tests peak power output (thus PPOSB and PPORBF) was defined as the highest work stage completed (the last work stage that was actually sustained for two minutes), and hence power output obtained. Constant load test with SB to determine ventilatory sensitivity: Ventilatory sensitivity was calculated from data obtained during 30 min cycle ergometery exercise at 50 W with a pedal cadence of 60 rpm. Participants started this test by breathing room air (initial 15 minutes) and then switched to breathing a humidified gas mixture containing 3% carbon dioxide, 21% oxygen and 76% nitrogen for a further 15 minutes (Kelley et al., 1982). The breathing mixture was directed to the inspiratory side of a Hans Rudolph respiratory valve (Hans Rudolph, Kansas City, Mo.). Ventilatory sensitivity was calculated using the following equation (Kelley et al., 1982): Constant load test: Following a 5 minute warm-up at 50 W participants completed a constant load cycle test with a pedal cadence of 60 rpm to volitional exhaustion on two separate occasions. On one occasion the peak power output achieved during the PPOSB test was adopted along with an SB breathing pattern. On another occasion the peak power output achieved during the PPORBF test was adopted along with an RBF breathing pattern. Time to complete each test (Tmax) was used to define the interval duration in the respective training programmes. Measurements during cycle ergometry tests: Participants breathed through a mouthpiece attached to a pneumotachograph during each cycle ergometry test. Expired air was sampled continuously by a metabolic cart(V-MAX29, SensorMedics Corporation, Yorba Linda, USA) for breath-by-breath determination of VE, VT, PETCO2, and the end-tidal partial pressure of oxygen (PETO2). The pneumotachograph and O2 and CO2 analysers were calibrated with a standard three-litre syringe and precision reference gases, respectively. For further statistical analysis breath-by-breath data were averaged for each 10 second interval. During the incremental exercise tests oxygen saturation (SpO2) was measured using a TruStatTM Pulse Oximeter (Datex-Ohmeda, Madison, USA). The pulse oximeter is an indirect oximetry measuring instrument, which displays SaO2 every 4 s. The ear probe was attached to the earlobe after cleaning the area with alcohol. Pulmonary function: A pneumotachograph spirometer (Vicatest P2a, Mijnhardt, Netherlands) was used to measure resting flow-volume profiles i.e. vital capacity (VC) and forced expiratory volume in one second (FEV1.0). Pulmonary function measurements were made according to European Respiratory Society recommendations (Miller et al., 2005).
Training protocolParticipants were randomly assigned to either the experimental group (Group E; n = 6) or the control group (Group C; n = 6). Both groups undertook three cycle ergometry interval training sessions per week for six weeks under supervision in the laboratory. Group E completed two to eight RBF training intervals per session. Interval durations were derived using manipulations of each participant’s Tmax value (between 60% and 75%) obtained during the constant load test with RBF (Laursen and Jenkins, 2002; Laursen et al., 2002). Interval intensities, however, were based on each participant’s PPORBF. Group C completed similar interval sets but did so with SB. Similar to Group E, interval durations ranged between 60% and 75% of each participant’s Tmax value which was obtained during the constant load test with SB. Once again, interval intensities were based on each participant’s PPOSB. A work-to-rest ratio of 2:1 or 1:1 was adopted; however, the resting periods between the intervals did not exceed five minutes. The number of intervals completed during each training session was set according to each participant’s ability to complete the prescribed training programme along with consideration of the duration of each interval and the resting period. Each training session lasted approximately 60 minutes. At the fifth (first mid testing), tenth (second mid testing) and fifteenth (third mid testing) training sessions, participants performed a further constant load test with the same breathing pattern as they used during training. The interval and rest durations and the number of intervals for subsequent training sessions were then adjusted to reflect each participant’s improved Tmax. The training programmes are summarized in Table 2. The study design is summarized in Figure 1.
Statistical analysesThe results are presented as means and standard deviations. Intra-group differences between the pre- and post-training values were calculated with a paired, two-tailed t-test. Analysis of covariance (ANCOVA), with pre-training values as covariates and post-training values as dependent variables, was used to test for differences between the groups resulting from different training interventions. Statistical significance was accepted at the p ≤0.05 level. Effect sizes were calculated using Cohen’s d statistics to assess the magnitude of the treatment with 0.2 deemed small, 0.5 medium and 0.8 large (Cohen, 1988). All statistical parameters were calculated using the statistics package SPSS (version 15.0, SPSS Inc., Chicago, USA) and the graphical statistics package Sigma Plot (version 9.0, Jandel, Tübingen, Germany).
All participants completed the prescribed training programme. The parameters which determined the intensity and duration of the training interval sets are shown in Table 3. According to the experimental protocol, the intensity of the interval sets was defined with the resistance of constant load tests with different breathing patterns (pre-training PPORBF for Group E and pre-training PPOSB for Group C) and was unchanged throughout the training. By contrast, the interval and rest durations and the number of intervals were adjusted after each mid testing according to each participant’s new Tmax value. As shown in Table 4, VC, ventilatory sensitivity and VO2 peak differed between groups in response to training (p < 0.05). A higher VC (p = 0.02, d = 0.36) and lower ventilatory sensitivity (p = 0.03, d = 0.81) were observed after training in Group E compared with pre-training values. By contrast, these parameters were unchanged in Group C. PPORBF was enhanced with training in both groups (p < 0.01, d=2.81 in Group E and p = 0.03, d = 0.66 in Group C; Figure 2(a)). Importantly, this enhancement was greater (for 275%) in Group E than in Group C (p < 0.01). PPOSB was increased with the training in Group C only (p < 0.01, d=2.02; Figure 2(b)). Respiratory parameters and SpO2 during the incremental test with RBF did not change (neither at submaximal intensities nor at PPORBF) throughout the training in Group C. On the other hand, there were significant training changes in these parameters in Group E. As shown in Figure 3 and 4, VE (p = 0.01, d = 2.4), VT (p = 0.01, d = 2.4), VO2 (p = 0.01, d = 3.2), VCO2 (p = 0.01, d=3.2) and PETco2 (p = 0.03, d = 0.86) obtained at PPORBF was higher post-training in Group E. In addition, there were significant differences in VE (p = 0.05), VT (p = 0.05) and VO2 (p = 0.04) obtained at post-training PPORBF between Group E and Group C. The lowest PPORBF obtained in pre- and post-training conditions was 150 W. Therefore, the average data of respiratory parameters and SpO2 measured to this work stage and at PPORBF during the incremental test with RBF for Group E only are presented in Figure 3 and 4. Respiratory parameters and SpO2 during the incremental test with SB did not change (neither at submaximal intensities nor at PPOSB) throughout the training in both groups. As shown in Table 4, only VO2 peak (obtained at the incremental test with SB) increased in Group C following training (p = 0.01, d = 1.05).
The present study demonstrated that training with RBF during cycle ergometry exercise not only increased VT in the face of RBF but also decreased ventilatory sensitivity in the presence of hypercapnia. Training with RBF also induced significantly larger improvement in PPORBF in Group E (42 ± 11%) compared with Group C (11 ± 9%; Figure 2(a)). Due to large (Group E) and medium (Group C) effect sizes in both tests, it could be suggested that the training intervention per se was primarily responsible for increased PPORBF. However, according to the training changes observed in spirometry parameters, ventilatory sensitivity, VO2 peak and VT at PPORBF, it does appear that training with different breathing patterns (i.e. spontaneous vs. RBF) induces specific adaptations. Given the necessity to coordinate breathing with stroke mechanics during front crawl swimming (Kapus et al., 2008; Town and VanNess, 1990), our findings are of particular relevance to this group of athletes.
Response to RBF during incremental exerciseWhen compared to spontaneously breathing exercise, cycle ergometry exercise with a similar reduction in breathing frequency (10 breaths per minute) is associated with a lower VE by a magnitude of 25% (Sharp et al., 1991) to 49% (Kapus et al., 2009; Kapus et al., 2010b; Yamamoto et al., 1987). Even greater reductions (55%) have been observed in the swimming literature where breathing frequency is reduced from breathing every second stroke cycle to breathing every sixth (Town and VanNess, 1990) or every eight (West et al., 2005) stroke cycle. As a reduction in VE is unlikely to be beneficial for exercise performance, it follows that an increase in VE (via VT) would be advantageous. Our results indicate that RBF training does increase VT during incremental exercise with RBF i.e. training reduced breathing restriction. Specifically, VT increased by 41 ± 19% following RBF and exercise training (Figure 3(b)). It is interesting that there were no significant differences between pre- and post-training VT measured at submaximal work stages. It seemed that the obtained levels of VT were sufficient to enable successful regulation of blood gases during submaximal exercise with RBF. In addition, these results indicated that the ventilatory level reached with RBF was a limiting factor only for maximal performance. Due to the prescribed and unchanged breathing frequency in the present study, an increase in VT was the only mechanism available for increasing VE (Figure 3(a)). This is consistent with reports that VT increases in proportion to the magnitude of breathing frequency restriction (Town and VanNess, 1990) as well as exercise intensity (Sharp et al., 1991). In addition to the observed increase in VT following RBF and exercise training, VC was also increased in response to RBF and exercise training by 8 ± 8% (Table 4). This could be due to an increase in inspiratory muscle force production (Clanton et al., 1987) as an anther possible effect of breathing with higher VT during training with RBF. Indeed, Jakovljevic and McConnell, 2009 found that inspiratory muscle fatigue was greater during front crawl swimming when breathing frequency was reduced from its already restricted pattern of breathing every second stroke cycle to breathing every fourth stroke cycle. They suggested that VT would increase to compensate and consequently inspiratory muscle work would rise for two reasons. Firstly, the elastic load of breathing increases as lung volume increases, and secondly, a functional weakening of inspiratory muscles occurs due to the relationship between lung volume, compliance and the length-tension characteristics (Rahn et al., 1946). Considering our observations and those of Jakovljevic and McConnell, 2009, it is possible that RBF training could have an effect on the strength of inspiratory muscles also. However, this suggestion requires further study.
Ventilatory response during exercise when breathing a 3% CO mixtureThe hypoventilation which occurs during RBF exercise is associated with a reduction in CO2 elimination. This increases the alveolar partial pressure of CO2 (Dicker et al., 1980; Holmér and Gullstrand, 1980), the fractional expired CO2 (West et al., 2005), PETco2 (Kapus et al., 2010a; 2010b), and the capillary blood partial pressure of CO2 (Kapus et al., 2009; 2010a; 2010b; Sharp et al., 1991; Yamamoto et al., 1987). In light of such findings it is not surprising that RBF training induces hypercapnic adaptations such that a higher level of CO2 can be tolerated i.e. a “hypercapnic training” effect (Dicker et al., 1980; Holmér and Gullstrand, 1980; Town and VanNess, 1990). Data from the current study supports this view. For example, participants in Group E showed an attenuated ventilatory sensitivity by 45 ± 27% (Table 4) during the hypercapnic exercise test, a response which was not observed in Group C. However, it should be emphasised that the hypercapnic stimulus was reducing throughout the training period. Considering that breathing restriction during RBF exercise was reducing due to training, it could be assumed that hypercapnic stimulus was higher in the first few training sessions and lower at end of the period of RBF training. Such an adaptation should reduce the urge to breath (Ferretti and Costa, 2003), which may partly explain why swimmers have been found to take fewer breaths during front crawl swimming following RBF training (Kapus et al., 2005). Increased tolerance to CO2 following RBF training is not restricted to swimmers, however. In activities where breath-holding occurs and thus so too does frequent exposure to hypercapnia, one would expect a blunted sensitivity to CO2. This is indeed the case in breath-hold divers (Delapille et al., 2001; Florio et al., 1979; Masuda et al., 1982) and under water hockey players (Davis et al., 1987; Lemaitre et al., 2007) as well as swimmers (Ohkuwa et al., 1980).
Response to SB during incremental exerciseDue to the additional stress caused by RBF, the workload during the interval training bouts in the current study was not identical between breathing conditions. Specifically, the workloads experienced by Group E were approximately 40% lower than in Group C (Table 3). These conditions might lead to different training effects during incremental exercise with SB (Figure 2(b)) and on VO2 peak (Table 4). According to the findings of Laursen, Shing, Peake, Coombes and Jenkins (2002) the interval training workloads adopted in the present study were high enough to increase VO2 peak during the SB condition i.e. Group C (Table 4) but were unlikely to in the RBF condition i.e. Group E. Furthermore, the increased VO2 peak in Group C might explain their improved PPORBF post-training (Figure 2(a)), even though VT was unchanged during exercise with RBF and when breathing a 3% CO2 mixture (Table 4). Possible study limitations: The present study has limitations. Firstly, because the participants were not blinded to the breathing intervention during training, one may argue that this might have influenced our results. However, participants were naäve and were not informed about the potential effect of training with different breathing patterns (i.e. spontaneous vs. RBF) on exercise performance. Furthermore, both trainings induced different, very specific adaptations. Therefore, the potential bias was minimised. Secondly, in previous studies CO2 sensitivity has been determined using the rebreathing method, which was first employed by Read, 1967, and the steady-state CO2 inhalation method (Kelley et al., 1982). The latter method provides two or three increments of inhaled CO2 and the composition of inhaled gas is adjusted by mixing compressed air with pure CO2 by using a precision gas bender. In the present study the ventilatory response to CO2 during exercise was determined by using only one predetermined gas mixture, which was previously mixed and analysed and provided from a high-pressure cylinder. This could be the reason for the higher absolute ventilatory sensitivity in the present study compared to data of previous studies using the steady-state CO2 inhalation method. The average ventilatory sensitivity values in the present study pre- and post-training were 4.7 l·min-1·mmHg-1 and 3.1 l·min-1·mmHg-1, respectively. These values are higher than the 3.25 l·min-1·mmHg-1 and 2.16 l·min-1·mmHg-1 obtained in divers and non-divers, respectively (Florio et al., 1979) but fall within the upper limit of those reported by McConnell and Semple, 1996. In addition, due to practical constraints, it was not possible to blind the participants to the start of the ventilatory sensitivity test. However, to minimise this influence, participants inhaled the breathing mixture for 15 minutes until VE and PETco2 were constant (Kelley et al., 1982). Only the data measured during the last three minutes were further analysed. Indeed, the data of both parameters were stable without additional noise during this period. Thirdly, it was not possible to standardize the training interventions between groups because of the demands associated with RBF. However, the design of the training programmes was based upon the established relationships of peak power output and Tmax on endurance performance (Laursen et al., 2002). Furthermore, both groups experienced similar and adequate levels of familiarisation. This was defined as cycling on the cycle ergometer and breathing in time with the metronome without problem for 15 minutes at a load of 100 W on at least two separate occasions. Consequently, it is unlikely that a learning effect accounted for the differences observed between groups. Indeed, substantial improvements in Tmax became evident after the ninth training session in Group E.
In conclusion, the effect of training with RBF during cycle ergometry exercise was twofold. It increased VT during incremental exercise where breathing frequency was restricted. In addition, RBF training decreased the ventilatory response to exercise when breathing a 3% CO2 mixture, which suggests an increased tolerance to CO2 following such training. These data are relevant to those athletes whose training or competitions involve hypoventilation such as swimmers. It could be suggested that swim training with RBF might permit swimmers to take fewer breaths and to hold their breath for longer, which would be of particular benefit during the underwater phases (flip turns, gliding, and underwater strokes) as well as providing an important biomechanical advantage (Pedersen and Kjendlie, 2003). However, training with RBF could not be realized during high velocity swimming due to the additional stress caused by such a breathing pattern. Therefore, it could be speculated that the combination of the two forms of intense front crawl training under different breathing conditions (swimming with RBF at a lower velocity, and swimming with the usual breathing frequency at higher velocities) would be beneficial for competitive swimmers.
ACKNOWLEDGEMENTS |
Research was supported by a grant from the Fundacija za šport, a foundation for financing sport organisations in Slovenia (RR-11-608). |
|
AUTHOR BIOGRAPHY |
 |
Jernej Kapus |
Employment: Assistant Professor, University of Ljubljana , Faculty of Sport |
Degree: PhD |
Research interests: Breathing during swimming, swimming with con tr olled breathing frequency, respiratory muscle tr aining, learning swimming |
E-mail: nejc.kapus@fsp.uni-lj.si |
|
 |
Anton Ušaj |
Employment: Professor, University of Ljubljana , Faculty of Sport |
Degree: PhD |
Research interests: Exercise physiology, acid-base regulation, carbohydrate metabolism, oxygen tr ansport to tissue |
E-mail: anton.usaj@fsp.uni-lj.si |
|
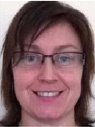 |
Mitch Lomax |
Employment: Senior Lecturer, Department of Sport & Exercise Science, University of Portsmouth , United Kingdom |
Degree: PhD |
Research interests: Swimming, impacts of respiratory muscle tr aining on performance, pulmonary limitations to exercise, circulatory and pulmonary interactions during exercise at sea level and in hypoxia |
E-mail: mitch.lomax@port.ac.uk |
|
|
|
REFERENCES |
 Clanton T.L., Dixon G.F., Drake J., Gadek J.E. ((1987)) Effects of swim training on lung volumes and inspiratory muscle conditioning. . Journal of Applied Physiology 62, 39-46.
|
 Cohen J. ((1988)) Statistical Power Analysis for the Behavioural Sciences. Hillsdale. Lawrence Erlbaum Associates.
|
 Cordain L., Stager J. ((1988)) Pulmonary structure and function in swimmers. . Sports Medicine 6, 271-278.
|
 Davis F.M., Graves M.P., Guy H.J., Prisk G.K., Tanner T.E. ((1987)) Carbon dioxide response and breath-hold times in underwater hockey players. . Undersea Biomedical Research 14, 527-534.
|
 Delapille P., Verin E., Tourny-Chollet C., Pasquis P. ((2001)) Ventilatory responses to hypercapnia in divers and non-divers: effects of posture and immersion. . European Journal of Applied Physiology 86, 97-103.
|
 Dicker S.G., Lofthus G.K., Thornton N.W., Brooks G.A. ((1980)) Respiratory and heart rate responses to controlled frequency breathing swimming. . Medicine and Science in Sports and Exercise 12, 20-23.
|
 Ferretti G., Costa M. ((2003)) Diversity in and adaptation to breath-hold diving in humans. . Comparative Biochemistry and Physiology 136, 205-213.
|
 Florio J.T., Morrison J.B., Butt W.S. ((1979)) Breathing pattern and ventilatory response to carbon dioxide in divers. . Journal of Applied Physiology 46, 1076-1080.
|
 Holmâ‹…r I., Gullstrand L. ((1980)) Physiological responses to swimming with a controlled frequency of breathing. . Scandinavian Journal of Sports Science 2, 1-6.
|
 Jakovljevic D.G., McConnell A.K. ((2009)) Influence of different breathing frequencies on the severity of inspiratory muscle fatigue induced by high-intensity front crawl swimming. . Journal of Strength and Conditioning Research 23, 1169-1174.
|
 Kapus J., Kapus V., Štrumbelj B., Ušaj A. ((2010a)) Can high intensity workloads be simulated at moderate intensities by reduced breathing frequency? . Biology of Sport 27, 163-168.
|
 Kapus J., Ušaj A., Kapus V. ((2010b)) Some metabolic responses to reduced breathing frequency during constant load exercise. . Medicina Sportiva 14, 13-18.
|
 Kapus J., Ušaj A., Kapus V., Štrumbelj B. ((2005)) The influence of training with reduced breathing frequency in front crawl swimming during a maximal 200 meters front crawl performance. . Kinesiologia Slovenica 11, 17-24.
|
 Kapus J., Ušaj A., Kapus V., Štrumbelj B. ((2009)) The difference in respiratory and blood gas values during recovery after exercise with spontaneous versus reduced breathing frequency. . Journal of Sports Science and Medicine 8, 452-457.
|
 Kapus J., Ušaj A., Kapus V., štrumbelj B. ((2002)) The influence of reduced breathing during front crawl swimming on some respiratory and metabolic values in blood. . Kinesiologia Slovenica 8, 14-18.
|
 Kapus J., Ušaj A., Kapus V., štrumbelj B. ((2003)) Influence of reduced breathing during intense front crawl swimming on some respiratory and metabolic values in blood. . Kinesiologia Slovenica 9, 12-17.
|
 Kapus J., Ušaj A., Štrumbelj B., Kapus V. ((2008)) Can blood gas and acid-base parameters at maximal 200 meters front crawl swimming be different between former competitive and recreational swimmers? . Journal of Sports Science and Medicine 7, 106-113.
|
 Kelley M.A., Owens G.R., Fishman A.P. ((1982)) Hypercapnic ventilation during exercise: effects of exercise methods and inhalation techniques. . Respiratory Physiology 50, 75-85.
|
 Laursen P.B., Jenkins D.G. ((2002)) The scientific basis for high-intensity interval training. . Sports Medicine 32, 53-73.
|
 Laursen P.B., Shing C.M., Peake J.M., Coombes J.S., Jenkins D.G. ((2002)) Interval training program optimization in highly trained endurance cyclists. . Medicine and Science in Sports and Exercise 34, 1801-1807.
|
 Lemaitre F., Polin D., Joulia F., Boutry A., Le Pessot D., Chollet D., Tourny-Chollet C. ((2007)) Physiological responses to repeated apneas in underwater hockey players and controls. . Undersea and Hyperbaric Medicine 34, 407-414.
|
 Maglischo E.W. ((2003)) Swimming fastest. Leeds. Human Kinetics.
|
 Masuda Y., Yoshida A., Hayashi F., Sasaki K., Honda Y. ((1982)) Attenuated ventilatory responses to hypercapnia and hypoxia in assisted breath-hold divers (Funado). . Japan Journal of Physiology 32, 327-336.
|
 Matheson G.O., McKenzie D.C. ((1988)) Breath holding during intense exercise: arterial blood gases, pH, and lactate. . Journal of Sports Medicine and Physical Fitness 64, 1947-1952.
|
 McConnell A.K., Semple E.S.G. ((1996)) Ventilatory sensitivity to carbon dioxide: the influence of exercise and athleticism. . Medicine and Science in Sports and Exercise 28, 685-691.
|
 Miller M.R., Hankinson J., Brusasco V., Burgos F., Casaburi R., Coates A., Crapo R., Enright P., van der Grinten C.P.M., Gustafsson P., Jensen R., Johnson D.C., MacIntyre N., McKay R., Navajas D., Pedersen O.F., Pellegrino R., Viegi G., Wanger J., Force A.E.T. ((2005)) Standardisation of spirometry. . European Respiratory Journal 26, 319-338.
|
 Ohkuwa T., Fujitsuka N., Utsuno T., Miyamura M. ((1980)) Ventilatory response to hypercapnia in sprint and long-distance swimmers. . European Journal of Applied Physiology 43, 235-241.
|
 Pedersen T., Kjendlie P.L. ((2003)) The effect of breathing action on velocity in front crawl swimming. . Portuguese Journal of Sport Sciences 6, 75-77.
|
 Peyrebrune M., Robinson J., Lakomy H., Nevill M., Chatard J.C. ((2003)) Effects of controlled frequency breathing on maximal tethered swimming performance. In: Proceedings of the 9th World Symposium of Biomechanics and Medicine in Swimming. Saint-Etienne: Laboratoire de Physiologie and Service de Exploration Fonctionnelle Cardio-respiratorie et de Medecine du Sport , 289-294.
|
 Rahn H., Otis A.B., Chadwick L.E., Wallace O.F. ((1946)) The pressure-volume diagram of the thorax and lung. . American Journal of Physiology 146, 161-178.
|
 Read D.J.C. ((1967)) A clinical method for assessing the ventilator response to carbon dioxide. . Australasian Annals of Medicine 16, 20-23.
|
 Sharp R.L., Williams D.J., Bevan L. ((1991)) Effects of controlled frequency breathing during exercise on blood gases and acid-base balance. . International Journal of Sports Medicine 12, 62-65.
|
 Town G.P., VanNess J.M. ((1990)) Metabolic responses to controlled frequency breathing in competitive swimmers. . Medicine and Science in Sports and Exercise 22, 112-116.
|
 West S.A., Drummond M.J., VanNess J.M., Ciccolella M.E. ((2005)) Blood lactate and metabolic responses to controlled frequency breathing during graded swimming. . Journal of Strength and Conditioning Research 19, 772-776.
|
 Yamamoto Y., Mutoh Y., Kobayashi H., Miyashita M. ((1987)) Effects of reduced frequency breathing on arterial hipoxemia during exercise. . European Journal of Applied Physiology 56, 522-527.
|
 Yamamoto Y., Takei Y., Mutoh Y., Miyashita M. ((1988)) Delayed appearance of blood lactate with reduced frequency breathing during exercise. . European Journal of Applied Physiology 57, 462-466.
|
|
|
|
|
|
|