|
|
|
ABSTRACT |
This study aimed to investigate the effects of 60-second static stretching on the neuromuscular control strategies of lower limb muscles during a squat jump (SJ), with a specific focus on changes in muscle synergy patterns, muscle weightings, and temporal activation characteristics. The muscles targeted for stretching included the quadriceps, hamstrings, and triceps surae. Electromyography (EMG) was used to assess the activity of the biceps femoris (BFL), triceps surae(TS), rectus femoris (RF), vastus lateralis (VL), and vastus medialis (VM). Twenty-five active males completed experiments under both a static stretching condition (SS) and a non-stretching condition (NS). Electromyography and non-negative matrix factorization (NMF) were employed to extract muscle synergy and the muscle weightings along with temporal activation characteristics were subsequently analyzed. The results revealed two distinct muscle synergy patterns in both the SS and NS. 60-second static stretching had no significant impact on the number of muscle synergy patterns during the squat jump. However, it significantly altered the contribution and temporal activation characteristics of individual muscles. Notably, post-stretching muscle activation levels were lower during the early phase of the jump, necessitating compensatory activation in the later phase to maintain performance. Additionally, jump heights were significantly lower in the stretched compared to the non-stretched condition.These findings suggest that while 60 seconds of static stretching before explosive movements may impair neuromuscular efficiency, ensuring proper and balanced static stretching for all muscle groups could help mitigate over-reliance on individual muscles. |
Key words:
Neuromuscular control, muscle synergy, electromyography, explosive performance
|
Key
Points
- Static stretching for 60 seconds did not alter the overall number of muscle synergies, but it significantly affected the contribution and activation timing of individual muscles during squat jumps, leading to compensatory activation in the later phases of the movement.
- The activation levels of specific muscles, particularly the vastus medialis, were significantly higher in the static stretching condition, suggesting compensatory activation due to reduced early-phase muscle activation following stretching.
- Temporal activation patterns were modified by static stretching, with the non-stretching condition showing greater activation during the early phase of the jump, while the static stretching condition exhibited higher activation in the later phase, potentially impacting performance outcomes.
- The study highlights the importance of considering the duration and intensity of static stretching in pre-exercise routines, as prolonged stretching may impair neuromuscular efficiency during explosive movements.
- Proper and balanced static stretching for all muscle groups could help mitigate over-reliance on individual muscles, thereby optimizing neuromuscular control strategies and potentially improving athletic performance.
|
Static stretching, a key component of pre-exercise routines, is commonly used to improve flexibility, enhance performance, and prevent injury(Reid et al., 2018; Zhu et al., 2022). However, extensive research over the past 25 years suggests that prolonged static stretching negatively affects performance, especially in strength- and power-based activities(Behm et al., 2016; Gurjão et al., 2009; Thomas et al., 2023). For example, Kay and Blazevich argue that holding static stretches for 60 seconds or longer can reduce muscle tension and neural drive, thereby inhibiting force output and explosive power (Kay and Blazevich, 2012), which prompt athletes and coaches to reconsider the suitability of static stretching during warm-up, especially its effects on neuromuscular control strategies. In complex motor tasks, muscle synergies are considered as crucial for achieving effective neuromuscular control(Mulla and Keir, 2023). Muscle synergies refer to the coordinated action of multiple muscle groups working together to complete specific tasks(Safavynia et al., 2011). This theory indicates that the nervous system simplifies motor control by assigning different weightings to muscles to form coordinated actions(Gentner and Classen, 2006). Research by Frère et al indicates that muscle synergies are relatively stable across various motor tasks contributing to improved movement efficiency and reduced injury risk(Frère and Hug, 2012). However, it remains unclear whether static stretching affects these muscle synergies, particularly in power-demanding movements like the squat jump (SJ)(Avloniti et al., 2016). Most existing studies have focused on the effects of static stretching on individual muscle force production and neural drive. Behm and Chaouachi found that static stretching significantly reduces muscle force output and reaction speed,impairing overall performance(Behm et al., 2011). Mulla and Keir further noted that the negative effects of static stretching are particularly pronounced when dynamic warm-up is absent, potentially leading to delayed muscle activation and reduced movement coordination(Behm et al., 2021). However, these studies merely confine to the examination physiological characteristics of single muscles or muscle groups without digging up the broader impact of static stretching on multi-muscle synergies. Neuromuscular control is a complex process involving the coordination of multiple muscles. In accordance with researches of Nishida et al, muscle synergies between different muscles can effectively reduce the complexity of motor control, enabling the central nervous system to achieve optimal coordination across multiple muscle groups(Nishida et al., 2017). Therefore, it will provide valuable insights into its impact on neuromuscular control strategies to investigate the effects of static stretching from the perspective of muscle synergies. The squat jump, a typical explosive movement, is widely applied in assess lower limb strength and neuromuscular coordination(La Torre et al., 2010; Li et al., 2024; Van Hooren and Zolotarjova, 2017). During the jump, multiple muscle groups must work synergistically to generate sufficient power and maintain stability throughout the movement. The formation of muscle synergies in such tasks relies not only on inter-muscular coordination but also on precise neural drive. However, it lacks thorough research on the potential impact of static stretching on muscle synergies during the squat jump. In light of this, this paper will initially apply static stretching to the analysis of muscle synergies. By comparing the differences in neuromuscular control strategies between the static stretching and the non-stretching during the squat jump, this paper will target at revealing the influence of static stretching on multi-muscle synergies. On the basis mentioned above, the study hypothesizes: Static stretching will not effectively alter the quantity of muscle synergies during the squat jump, but may transform the relative contribution and activation timing of individual muscles within the synergies.
Study populationA total of 25 highly active males (a priori power analysis: α = 0.05, β = 0.8; mean age: 22.5 ± 1.3 years; height: 177.3 ± 5.4 cm; weight: 68.2 ± 7.1 kg) were recruited for this study. Given the non-linear nature of the muscle synergy data, traditional power analysis using G*Power was not deemed appropriate. Instead, the sample size was determined based on a combination of practical considerations and previous research findings in the field of muscle synergies. Specifically, a minimum sample size of 24 participants was chosen to ensure sufficient statistical power to detect significant differences in muscle synergies, as suggested by similar studies (Frère and Hug, 2012; Gentner and Classen, 2006). To further enhance the robustness of the study, the sample size was increased to 25 participants. None of the participants had a history of lower limb injury or neurological disease. Each participant completed experiments under both a static stretching condition and a non-stretching condition. SS performed 60 seconds of stretching of lower extremity muscles, including the quadriceps, hamstrings, and triceps surae, prior to jumping. All subjects signed an informed consent form before participating in the experiment, which was approved by the Ethics Committee of Jeonbuk National University (JBNU2024-09-015-002).
Study designThis study employed a randomized crossover experimental design, in which experiments were conducted under both static stretching and no stretching conditions. Each subject performed five repetitive Squat Jump (SJ) trials in each experimental condition. The order of the experiments was determined by random draw to ensure reliability and fairness of the results. The SJ trials were divided into distinct take-off phases, and neuromuscular control strategies were analyzed under varying conditions by acquiring electromyography (EMG) signals and kinematic data. The phases of the SJ jumps were delineated using Visual 3D biomechanical analysis software, with differentiation criteria as follows: E1 refers to the preparation for the movement; E2 spans from the initiation of the movement until the athlete achieves a knee flexion angle of 90; E3 represents the peak jump height during the knee's transition from flexion to extension. The aforementioned criteria constitute the complete SJ jump take-off phases test.
Experimental preparationAll evaluations were performed using a 3D motion tracking system equipped with 13 infrared cameras (Prime 17 W, OptiTrack, Natural Point, Inc., Corvallis, Oregon, USA), with the capture rate set to 100 Hz. 14-mm-diameter reflective markers were strategically placed at key anatomical points on the participants' lower limbs, for a total of 20 markers. Ground reaction forces were recorded at a sampling frequency of 1200 Hz using an OR6-6-2000 force platform (Advanced Mechanical Technology, Inc., Watertown, MA, USA). The electrical activity of the biceps biceps femoris (BFL), triceps surae(TS), rectus femoris (RF), vastus lateralis (VL) and vastus medialis (VM) muscles of the main leg was recorded by an EMG device (Trigno Avanti Sensor, Delsys, Natick, MA, USA) at a sampling rate of 1200 Hz. Electrodes were placed at the mid-belly of each muscle following SENIAM guidelines, with inter-electrode spacing of 10 mm and aligned parallel to muscle fiber orientation to ensure accurate signal acquisition. Each Trigno Avanti Sensor (37 mm × 27 mm × 13 mm, 14 g) consisted of a dual differential EMG sensor with silver-striped electrodes (5 mm × 1 mm, inter-electrode distance: 10 mm), a common mode rejection ratio of 80 dB, an amplifier gain of 909, and an analog Butterworth filter with a bandwidth of 20-450 Hz. Prior to placing the electrodes, the corresponding skin sites were shaved with an abrasive gel and then cleaned with alcohol. Synchronization data were collected from the motion capture system, force platform and EMG system using Motive 2.2.0 software (OptiTrack, Natural Point, Inc., Corvallis, OR, USA).
Test programAll participants underwent a 5-minute dynamic warm-up on a motorized bicycle (Swedish Monark 894E Wingate test bike ergometer) at 60 rpm and 1kp (70 W) resistance. After the warm-up, a 5-minute rest period was allotted to allow body temperature and oxygen consumption to return to normal(Bishop, 2003). The SJ jump test was conducted 3 minutes after the completion of the static stretching intervention. The static stretching intervention targeted the quadriceps, gastrocnemius, and hamstring muscles, while a control group received no stretching. A washout period of more than 24 hours was implemented between sessions to avoid baseline effects(Hopkins, 2000).
InterventionDuring static stretching, the target muscles (gastrocnemius, hamstrings, and quadriceps) were stretched in a randomized order. The selection of these muscles was based on previous studies(La Torre et al., 2010; Li et al., 2023). The static stretching protocol, based on Chen et al.(Chen et al., 2023; Reid et al., 2018), involved two repetitions of the static stretching exercise for each muscle group of each leg, with each exercise lasting 30 seconds interspersed with a 15-second passive rest period, for a total cumulative stretching time of 60 seconds for each muscle group. The stretches were calibrated to a threshold of discomfort but did not result in pain. Subsequently, active quadriceps and hamstring stretches were performed in an upright position. For the quadriceps, participants leaned one hand against a wall and bent the knee of the target leg to 90°for balance. The ipsilateral hand grasps the ankle and gently pulls the heel toward the gluteus area to ensure proper extension. Hamstrings stretches are performed by placing one leg on a step with the knee slightly bent and leaning forward from the hip until a stretch is felt. The triceps surae stretch involves the participant in the supine position with the experimenter assisting with foot dorsiflexion to achieve the desired stretch.
SJ 90° jump testIn the SJ90° jump test, participants were instructed to start from a standing position on the force plate in a preparatory stance. They then flexed their knees to a 90° angle and initiated the jump from this position, emphasizing explosive power during the take-off phase to reach the maximum height (Figure 1). Prior to the actual test, participants completed a practice jump to become familiar with the procedures. To standardize the initial position, participants stood in the designated jump area with their feet shoulder-width apart, hands positioned in front of their chest, and knees flexed to 90 degrees. During the jump, participants were instructed to maintain their hands in front of their chest to minimize the influence of arm swing on jump performance. After holding the 90°squat position for approximately one second, participants were directed to jump vertically as quickly and forcefully as possible. This setup was designed to reduce the contribution of elastic muscle elements and reflex responses in jump performance (Bojsen-Møller et al., 2005). For temporal activation analysis, the percentage phases of the squat jump were defined as follows: 0-18% represents the isometric holding phase (participants maintained a 90° knee flexion position as a static preparation phase); 18%-40% corresponds to the initial push-off phase (transition from static position to upward thrust); 40%-85% represents the maximal push-off phase (primary force generation and takeoff); and 85%-100% represents the flight phase (body completely airborne after takeoff).
Data processing and analysisEMG data from each conditions jump phase intercepted according to kinematic stages were imported into Python for processing. The EMG signals were band-pass filtered (10-500 Hz, Butterworth filter, 4th order), The root mean square (RMS) with a 10 ms window was applied to smooth the EMG signals (Santos et al., 2012; St-Amant et al., 1998). Electromyography signals were normalized using electro myography signals from a maximal voluntary contraction (MVC) as a reference. Three different experiments for MVC were executed for each separate muscle, with 1min rest between examinations.MVC tests were conducted using standardized isometric contractions: for the vastus medialis (VM) and vastus lateralis (VL), participants performed a maximal knee extension against resistance; for the rectus femoris (RF), participants executed a maximal hip flexion against resistance; for the biceps femoris (BFL), participants performed a maximal knee flexion against resistance; and for the triceps surae(TS), participants executed a maximal plantar flexion while seated with resistance applied to the foot. The experiments were performed with the segments positioned in that each muscle has an intervention as preferential agonist developing its maximal intensity of activation (VencesBrito et al., 2011). After doing the same RMS processing on the collected MVC data, the collected experimental data was normalized using MVC data. For each subject and the two conditions (intervention and non-intervention), the two datasets with the highest correlations (where all muscle correlations were greater than 0.9) were selected for co-extraction (Aoyama et al., 2022). Non-negative matrix factorization (NMF) was ap plied to decompose the pre-processed EMG signal matrix to decompose and derive muscle synergies. The algorithm mic formulation is as follows(Lee and Seung, 1999; Russo et al., 2024). The EMG matrix is an initial matrix of D rows and T columns (T = 5 is the number of muscles, and D = 100 is the number of normalized time points). W is a matrix comprising D rows and N columns (N is the number of muscle synergies), and H is a matrix comprising N rows and T columns. E is defined as the difference between the initial and reconstructed EMG matrices. The algorithm is based on it relatively updating the initial random guesses for H and W, converging to a locally optimal matrix decomposition. The algorithm is repeated at least 20 times for each subject to avoid local optimal solutions. The overall optimal solution (i.e., minimizing the squared error between the initial and reconstructed EMG patterns) was retained. Using the algorithm, the pre-processed EMG matrices for each subject’s two conditions jump were decom posed into muscle synergy weighted (W) and activated (H) matrices based on temporal order. The term ‘motor module’ refers to the muscle synergy weighted matrix (W), whereas ‘motor primitives’ refers to the activation matrix (H). Each motor module is represented as an (N x T) vector, with its components reflecting the relative contribution (weight) of each muscle to that specific synergy, and the corresponding motor primitives indicate the module’s activation patterns over the time series. There are two common approaches for determining the number of synergies, both requiring the variance accounted for (VAF) to be calculated first (Figure 2). The first approach is to choose the number of synergies that account for more than 0.9 of the VAF (Torres-Oviedo and Ting, 2010),whereas the second approach uses the inflection point of the best linear fit result as the criterion(Clark et al., 2010). The average total VAF was defined as follow : To determine the number of muscle synergies, VAF from 1 to 9 was calculated based on the number of muscles sampled. And, VAF two conditions jump for each subject at each number of synergies was calculated to ensure that the muscle synergies accurately explained the neuro muscular control strategy at two conditions jump for each subject. When the motor module was 2, the ASS group’s VAF = 0.965 ± 0 .030, and the CON group’s VAF = 0.969 ± 0.030. As a result, we limited the number of synergistic elements (N) to 2 and extracted the motor modules and motor primitives for muscle synergism when subjects two conditions jump. The k-means clustering algorithm was utilized to classify the motor modules retrieved from each jump for the 25 subjects. Since VAF indicates that there are 2 motor modules, K was set to 2. Considering that the initial clustering center of mass influences the k-means solution, randomness was reduced by repeating the process 50 times with different initial centers of mass. Profile scores were computed for all clustering out comes to assess the optimal clustering result. The result with the highest profile score was selected as the optimal clustering outcome, and motor modules with their corresponding motor primitives were outputted. Each conditions jump was clustered using this approach, and the motor modules and motor primitives for all conditions were obtained.
Statistical analysisTo investigate the effects of static stretching on neuromuscular control strategies during the jump phase, this study employed paired-sample t-tests and Statistical Parametric Mapping (SPM) to analyze muscle weightings and temporal activation characteristics. Prior to conducting the paired-sample t-tests, the normality of the data was assessed using a normal distribution test. If the data did not meet the normality assumption, a nonparametric test was utilized instead. Cohen’s d effect sizes were calculated to quantify the magnitude of the differences, with descriptors defined as follows: trivial (d < 0.2), small (0.2 ≤ d < 0.5), moderate (0.5 ≤ d < 0.8), and large (d ≥ 0.8). Additionally, paired-sample t-tests were conducted to analyze differences in jump height. The level of statistical significance was set at p < 0.05 for all tests. All statistical analyses were performed using PyCharm Community Edition 2024.2.3.
The movement modules during the take-off phase for all participants in both groups are illustrated in Figure 3. Each group demonstrated two distinct movement modules, with specific dominant muscles associated with the take-off phase. In movement module 1 (M1), paired t-tests on the muscle weightings indicated no significant differences in the weightings of the biceps femoris, triceps surae, rectus femoris, or vastus lateralis between the two groups (Figure 4). The vastus medialis weighting in the SS was significantly higher than that in the NS (p < 0.05, d = 1.223). In movement module 2, no significant differences were observed in muscle weightings between the groups (p > 0.05); however, the overall muscle weightings in the NS were greater than those in the SS (Figure 5).
Characteristics of movement primitivesIn movement primitive 1, the activation level in the NS during the 18%-40% phase was significantly higher than that in the SS (p < 0.001, d = 1.127). Conversely, during the 85% - 100% phase, the SS exhibited significantly higher activation levels compared to the NS (p < 0.001, d = 1.329) (Figure 6). In movement primitive 2, the SS demonstrated significantly lower activation only during the 75%-100% phase compared to the NS (p < 0.05, d = 0.846). Additionally, jump heights were significantly lower in the stretched compared to the non-stretched condition (p < 0.05, d = 0.65).
This study investigated the effects of 60 seconds of static stretching on neuromuscular control strategies during the deep squat jump (SJ) in active male adults, specifically comparing the stretching with the non-stretching. The analysis of muscle synergistic patterns revealed that both groups exhibited two stable muscle synergistic patterns during jumping, with the number of synergies remaining consistent across both groups. This finding aligns with Frère et al.'s study, which noted that even when subjected to specific interventions such as static stretching, the stability of muscle synergy patterns remains high across motor tasks, and the quantity of muscle synergy does not change readily(Frère and Hug, 2012). Further synergistic decomposition results indicated that the two motor modules were activated sequentially and reached their peaks at different times. Specifically, the primary muscle contributions in Model 1 were from the vastus medialis, vastus lateralis, and rectus femoris muscles, while the triceps surae muscle exhibited the highest contribution in Model 2. Notably, all muscles in the NS demonstrated greater contributions than those in the SS. This finding aligns with the research conducted by Kay and Young, which suggested that static stretching may result in a decrease in muscle activation levels, thereby impacting overall athletic performance(Kay and Blazevich, 2012; Montalvo et al., 2022; Young et al., 2001).Reduced muscle tone and neural drive following static stretching may have been the primary reason for the diminished contribution of each muscle in the SS, particularly in mode 2(Bradley et al., 2007; Hough et al., 2009). This study represents the first exploration of the effects of static stretching on the synergistic activation of multiple muscle groups through the framework of muscle synergy patterns, thereby addressing a significant gap in this area of research. The results of the present study align with those of Nishida et al, who observed that the overall number of synergistic patterns remained stable across various motor tasks (e.g., changes in muscle activation strategies during running)(Nishida et al., 2017). However, the degree of contribution of specific tasks to individual muscles was influenced by these variations. Static stretching may have modified the contribution of certain muscles, particularly in mode 2, where the muscles in the NS generally exhibited greater contributions than those in the SS. This finding suggests that unstretched muscles maintain a higher neural drive, resulting in a more balanced activation pattern(Yamaguchi et al., 2006). In Mode 1, the contribution of the vastus medialis femoris was significantly higher in the SS compared to the NS, which contradicts the findings of Yamaguchi et al(Yamaguchi et al., 2006), who demonstrated that static stretching typically inhibits the activation of the quadriceps. However, the increased VM contribution observed in the SS in this study may be attributed to the lower activation level at the onset of the jump following static stretching, which led to compensatory activation of the muscle at a later stage. This is reflected in the temporal activation profile of the H-matrix, which indicates that the activation level of the SS was significantly higher than that of the NS during the 85% - 100% phase of Mode 1. This compensatory activation may result from a reduced neural drive associated with static stretching, leading the VM to assume a greater load to compensate for the diminished contribution of other muscles. This phenomenon aligns with the neural theory proposed by Todorov et al, which posits that specific muscles are regulated by the nervous system to sustain task performance through compensatory activation(Todorov and Jordan, 2002). The significant activation of the VM in the SS can be interpreted as a compensatory response of the central nervous system to static stretch disturbances. Regarding the temporal activation characteristics, SPM analysis indicated that the NS in Mode 1 exhibited significantly higher activation levels than the SS during the 18%-40% phase. This finding suggests that the unstretched muscles were capable of activating earlier and with greater intensity in the initial phase of the jump. This may be attributed to the fact that muscles that have not undergone static stretching exhibit higher neural drive and greater muscle elasticity, resulting in a more efficient power output during the initial phases of the jump(Gesel et al., 2022).Power et al showed that static stretching significantly reduced jumping and strength performance over a short period of time, which is consistent with the findings of this study(Power et al., 2004). In contrast, the SS demonstrated a higher level of activation during the 85% - 100% phase, likely as a compensatory mechanism for the reduced activation observed at the jump's onset. This phenomenon can be elucidated by the findings of Fowles et al, which indicate that muscles subjected to static stretching require a longer duration to return to optimal force output, thereby exhibiting compensatory activation later in the activity(Fowles et al., 2000). In Mode 2, the activation level of the NS during the 75% - 100% phase was significantly higher than that of the SS, further supporting the hypothesis that static stretching may reduce neural activation efficacy. This finding aligns with the research of Behm and Chaouachi, which indicates that static stretching results in a short-term decrease in power output and reaction speed(Behm and Chaouachi, 2011). Although no significant difference in muscle contribution was observed between the two groups in Mode 2, the NS demonstrated higher weights per muscle compared to the SS, highlighting the inhibitory effect of static stretching on overall muscle activation. Matsuo et al. study further supports the effect of static stretching on muscle tone and elasticity, which may lead to a reduction in neural actuation(Nakamura et al., 2013). Static stretching, by decreasing muscle elasticity and neural sensitivity, may lead to higher activation levels being required later in the task to achieve the desired outcome(Sasajima and Kubo, 2024). The study by Fan et al, although not focused on static stretching, found that various tasks in basketball elicited flexible changes in muscle synergy patterns(Fan et al., 2024). This indicates that the nervous system optimizes motor performance by modulating muscle contributions and temporal activation. Consistent with the findings of the present study, the neuromuscular system may compensate for the initial lack of activation following static stretching by enhancing activation at a later stage of the jump.
Research limitationsThis study analyzed only five major lower limb muscles, specifically the vastus medialis, vastus lateralis, rectus femoris, biceps femoris, and . triceps surae. Therefore, future research should include additional muscles to achieve a more comprehensive understanding of neuromuscular synergy patterns. This study exclusively included male college athletes, which limits the generalizability of the findings to female populations, various age groups, and non-athlete cohorts. Variations in gender, age, and training background may significantly impact the effects of static stretching on neuromuscular control strategies. Future research should incorporate subjects of diverse genders, age groups, and training backgrounds to investigate the influence of these variables on muscle synergy patterns. This study examined the effects of 60 seconds of static stretching, without addressing the impact of varying durations or intensities of stretching. The duration and intensity of static stretching may influence muscle synergy patterns differently. Future research should investigate the effects of static stretching at various durations (e.g., 30 seconds, 120 seconds) and intensities on neuromuscular control to enhance the formulation of effective stretching strategies for athletes and coaches.
Practical application
1) Optimizing exercise preparation strategiesThe present study demonstrates that while static stretching does not alter the overall number of muscle synergy patterns, it significantly affects the contribution and temporal activation characteristics of individual muscles. This finding suggests that athletes should exercise caution when using prolonged static stretching prior to high-intensity, explosive exercise. Depending on the specific athletic task, coaches may consider incorporating dynamic warm-ups or short static stretches to ensure that muscles are activated quickly and efficiently during jumps or other explosive maneuvers, thereby avoiding delayed activation or loss of strength in the early stages of the jump.
2) Develop a personalized stretching programStatic stretching does not uniformly affect different muscle groups. Research has shown that the vastus medialis femoris muscle demonstrates compensatory activation following static stretching, indicating that, in certain instances, static stretching may cause specific muscles to bear an increased load. Consequently, coaches and rehabilitators should consider individual muscle adaptations when designing a stretching program, paying particular attention to the duration and intensity of the stretching.
3) Improve muscle activation homeostasisThe results of the present study suggest that unstretched muscles can participate in exercise more effectively, particularly at the onset of a jump, where they exhibit higher levels of activation. In light of these findings, athletes and coaches should ensure that each muscle is adequately stretched during static stretching. This practice will enhance the balance of the stretching technique and help prevent the performance decline associated with the unbalanced activation of individual muscles.
ACKNOWLEDGEMENTS |
The author reports no actual or potential conflicts of interest. While the datasets generated and analyzed in this study are not publicly available, they can be obtained from the corresponding author upon reasonable request. All experimental procedures were conducted in compliance with the relevant legal and ethical standards of the country where the study was carried out. |
|
AUTHOR BIOGRAPHY |
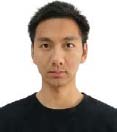 |
Weishuai Guo |
Employment: A lecturer at Pindingshan University. |
Degree: PhD |
Research interests: Acute training or warming-up effects on muscular performance and strength development. |
E-mail: g15238279199@163.com |
|
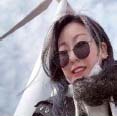 |
Youngsuk Kim |
Employment: A lecturer at Pindingshan University. |
Degree: PhD |
Research interests: Human muscular coordination and control mechanism for physical activities such as basketball, badminton, volleyball, and running. |
E-mail: ys43530@jbnu.ac.kr |
|
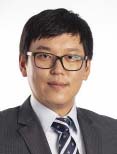 |
Junsig Wang |
Employment: Ass. Prof. at Kyung Hee University. |
Degree: PhD |
Research interests: Neuromuscular control mechanism of gait in special populations. |
E-mail: jwang@khu.ac.kr |
|
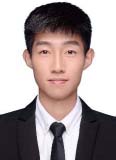 |
Tengfei Dong |
Employment: A post-doc fellow of Kinesiology laboratory at Jeonbuk National University. |
Degree: PhD |
Research interests: Human neuromuscular coordination patterns and injury mechanism during landings for badaminton and running. |
E-mail: dongtf@jbnu.ac.kr |
|
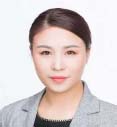 |
Xuan Tang |
Employment: A lecturer at Yunnan University. |
Degree: PhD |
Research interests: The effects of dance sports on slips, trips, and falls. |
E-mail: tangxuan@ynu.edu.cn |
|
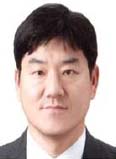 |
Sukwon Kim |
Employment: Prof. and the director of Kinesiology laboratory at Jeonbuk National University. |
Degree: PhD |
Research interests: Human muscular coordination and control mechanism for physical activities such as soccer, basketball, badminton, volleyball, tennis, gymnastics, and running. |
E-mail: rockwall@jbnu.ac.kr |
|
|
|
REFERENCES |
 Aoyama T., Ae K., Kohno Y. (2022) Interindividual differences in upper limb muscle synergies during baseball throwing motion in male college baseball players. Journal of Sports Science 145, 111384-. Crossref
|
 Avloniti A., Chatzinikolaou A., Fatouros I.G., Avloniti C., Protopapa M., Draganidis D., Stampoulis T., Leontsini D., Mavropalias G., Gounelas G. (2016) The acute effects of static stretching on speed and agility performance depend on stretch duration and conditioning level. Journal of Strength and Conditioning Research 30, 2767-2773. Crossref
|
 Behm D.G., Blazevich A.J., Kay A.D., McHugh M. (2016) Acute effects of muscle stretching on physical performance, range of motion, and injury incidence in healthy active individuals: A systematic review. Sports Medicine 41, 1-11. Crossref
|
 Behm D.G., Chaouachi A. (2011) A review of the acute effects of static and dynamic stretching on performance. Medicine and Science in Sports and Exercise 111, 2633-2651. Crossref
|
 Behm D.G., Kay A.D., Trajano G.S., Blazevich A. (2021) Mechanisms underlying performance impairments following prolonged static stretching without a comprehensive warm-up. Journal of Sports Science 121, 67-94. Crossref
|
 Bishop D. (2003) Warm up II: Performance changes following active warm up and how to structure the warm up. Sports Medicine 33, 483-498. Crossref
|
 Bojsen-Møller J., Magnusson S.P., Rasmussen L.R., Kjaer M., Aagaard P. (2005) Muscle performance during maximal isometric and dynamic contractions is influenced by the stiffness of the tendinous structures. Journal of Physiology 99, 986-994. Crossref
|
 Bradley P.S., Olsen P.D., Portas M.D. (2007) The effect of static, ballistic, and proprioceptive neuromuscular facilitation stretching on vertical jump performance. Journal of Strength and Conditioning Research 21, 223-226. Crossref
|
 Chen C.H., Chiu C.H., Tseng W.C., Wu C.Y., Su H.H., Chang C.K., Ye X. (2023) Acute effects of combining dynamic stretching and vibration foam rolling warm-up on lower-limb muscle performance and functions in female handball players. Journal of Sports Science and Medicine 37, 1277-1283. Crossref
|
 Clark D.J., Ting L.H., Zajac F.E., Neptune R.R., Kautz S.A. (2010) Merging of healthy motor modules predicts reduced locomotor performance and muscle coordination complexity post-stroke. Journal of Neurophysiology 103, 844-857. Crossref
|
 Fan P., Yang Z., Wang T., Li J., Kim Y., Kim S. (2024) Neuromuscular control strategies in basketball shooting: Distance-dependent analysis of muscle synergies. Journal of Sports Science and Medicine 23, 571-580. Crossref
|
 Fowles J.R., Sale D.G., MacDougall J.D. (2000) Reduced strength after passive stretch of the human plantarflexors. Journal of Applied Physiology 89, 1179-1188. Crossref
|
 Frère J., Hug F. (2012) Between-subject variability of muscle synergies during a complex motor skill. Journal of Experimental Biology 6, 99-. Crossref
|
 Gentner R., Classen J. (2006) Modular organization of finger movements by the human central nervous system. Journal of Neuroscience 52, 731-742. Crossref
|
 Gesel F.J., Morenz E.K., Cleary C.J., LaRoche D.P. (2022) Acute effects of static and ballistic stretching on muscle-tendon unit stiffness, work absorption, strength, power, and vertical jump performance. Journal of Sports Science 36, 2147-2155. Crossref
|
 Gurjão A.L., GonÇalves R., de Moura R.F., Gobbi S. (2009) Acute effect of static stretching on rate of force development and maximal voluntary contraction in older women. Journal of Aging and Physical Activity 23, 2149-2154. Crossref
|
 Hopkins W.G. (2000) Measures of reliability in sports medicine and science. Sports Medicine 30, 1-15. Crossref
|
 Hough P.A., Ross E.Z., Howatson G. (2009) Effects of dynamic and static stretching on vertical jump performance and electromyographic activity. Journal of Strength and Conditioning Research 23, 507-512. Crossref
|
 Kay A.D., Blazevich A. (2012) Effect of acute static stretch on maximal muscle performance: A systematic review. Medicine and Science in Sports and Exercise 44, 154-164. Crossref
|
 La Torre A., Castagna C., Gervasoni E., Cè E., Rampichini S., Ferrarin M., Merati G. (2010) Acute effects of static stretching on squat jump performance at different knee starting angles. Journal of Strength and Conditioning Research 24, 687-694. Crossref
|
 Lee D.D., Seung H.S. (1999) Learning the parts of objects by non-negative matrix factorization. Nature 401, 788-791. Crossref
|
 Li M., Kim Y., Guo W., Fan P., Wang J., Kim S. (2024) Effects of conditioning contractions on lower-body explosive force post-static stretching. International Journal of Sports Medicine 23, 571-580.
|
 Li M., Meng X., Guan L., Kim Y., Kim S. (2023) Comparing the effects of static stretching alone and in combination with post-activation performance enhancement on squat jump performance at different knee starting angles. Journal of Sports Science and Medicine 22, 769-. Crossref
|
 Montalvo S., Gonzalez M.P., Dietze-Hermosa M.S., Martinez A., Rodriguez S., Gomez M., Cubillos N., Ibarra-Mejia G., Tan E., Dorgo S. (2022) Effects of different stretching modalities on the antagonist and agonist muscles on isokinetic strength and vertical jump performance in young men. Journal of Strength and Conditioning Research 39, 173-183. Crossref
|
 Mulla D.M., Keir P. (2023) Neuromuscular control: From a biomechanist's perspective. Journal of Biomechanics 5, 1217009-. Crossref
|
 Nakamura M., Ikezoe T., Takeno Y., Ichihashi N. (2013) Time course of changes in passive properties of the gastrocnemius muscle–tendon unit during 5 min of static stretching. Journal of Applied Physiology 18, 211-215. Crossref
|
 Nishida K., Hagio S., Kibushi B., Moritani T., Kouzaki M. (2017) Comparison of muscle synergies for running between different foot strike patterns. Plos One 12, e0171535-. Crossref
|
 Power K., Behm D., Cahill F., Carroll M., Young W. (2004) An acute bout of static stretching: Effects on force and jumping performance. Journal of Sports Science 36, 1389-1396. Crossref
|
 Reid J.C., Greene R., Young J.D., Hodgson D.D., Blazevich A.J., Behm D.G. (2018) The effects of different durations of static stretching within a comprehensive warm-up on voluntary and evoked contractile properties. European Journal of Applied Physiology 118, 1427-1445. Crossref
|
 Russo M., Scano A., Brambilla C., d'Avella A. (2024) SynergyAnalyzer: A Matlab toolbox implementing mixed-matrix factorization to identify kinematic-muscular synergies. Journal of Biomechanics 251, 108217-. Crossref
|
 Safavynia S., Torres-Oviedo G., Ting L. (2011) Muscle synergies: Implications for clinical evaluation and rehabilitation of movement. Journal of Neurophysiology 17, 16-24. Crossref
|
 Santos A.B., Soares D.P., Candotti C.T. (2012) Smoothing EMG signals: Implications on delay calculation. Journal of Electromyography and Kinesiology 12, 60-72. Crossref
|
 Sasajima S., Kubo K. (2024) Effect of static stretching on tendon hysteresis and efficiency during repetitive jumping. Journal of Sports Science 38, 1041-1047. Crossref
|
 St-Amant Y., Rancourt D., Clancy E.A. (1998) Influence of smoothing window length on electromyogram amplitude estimates. Journal of Electromyography and Kinesiology 45, 795-799. Crossref
|
 Thomas E., Ficarra S., Nunes J.P., Paoli A., Bellafiore M., Palma A., Bianco A. (2023) Does stretching training influence muscular strength? A systematic review with meta-analysis and meta-regression. Journal of Strength and Conditioning Research 37, 1145-1156. Crossref
|
 Todorov E., Jordan M.I. (2002) Optimal feedback control as a theory of motor coordination. Nature Neuroscience 5, 1226-1235. Crossref
|
 Torres-Oviedo G., Ting L.H. (2010) Subject-specific muscle synergies in human balance control are consistent across different biomechanical contexts. Journal of Neurophysiology 103, 3084-3098. Crossref
|
 Van Hooren B., Zolotarjova J. (2017) The difference between countermovement and squat jump performances: A review of underlying mechanisms with practical applications. Sports Medicine 31, 2011-2020. Crossref
|
 VencesBrito A.M., Ferreira M.A.R., Cortes N., Fernandes O., Pezarat-Correia P. (2011) Kinematic and electromyographic analyses of a karate punch. Journal of Sports Science 21, 1023-1029. Crossref
|
 Yamaguchi T., Ishii K., Yamanaka M., Yasuda K. (2006) Acute effect of static stretching on power output during concentric dynamic constant external resistance leg extension. Journal of Strength and Conditioning Research 20, 804-810. Crossref
|
 Young W., Elliott S. (2001) Acute effects of static stretching, proprioceptive neuromuscular facilitation stretching, and maximum voluntary contractions on explosive force production and jumping performance. Journal of Sports Science 72, 273-279. Crossref
|
 Zhu Y., Feng Y., Huang F., Li Y., Wang W., Wang X., Cao X., Zhang Z. (2022) Changes in stiffness of the specific regions of knee extensor mechanism after static stretching. Journal of Sports Science 10, 958242-. Crossref
|
|
|
|
|
|
|