|
|
|
ABSTRACT |
Living high-training low (LHTL) is performed by competitive athletes expecting to improve their performance in competitions at sea level. However, the beneficial effects of LHTL remain controversial. We sought to investigate whether 21 days of LHTL performed at a 3,000 m simulated altitude (fraction of inspired oxygen [FIO2]=14.5%) and at sea level can improve hematological parameters, exercise economy and metabolism, hemodynamic function, and exercise performance compared with living low-training low (LLTL) among competitive athletes. All participants (age = 23.5 ± 2.1 years, maximal oxygen consumption [VO2max] = 55.6 ± 2.5 mL·kg-1·min-1, 3,000 m time trial performance=583.7 ± 22.9 seconds) were randomly assigned to undergo LHTL (n = 12) or LLTL (n = 12) and evaluated before and after the 21 days of intervention. During the 21-day intervention period, the weekly routine for all athletes included 6-day training and 1-day rest. The daily training programs consisted of >4 hours of various exercise programs (i.e., jogging, high-speed running, interval running, and 3,000 m or 5,000-m time trial). The LHTL group resided in a simulated environmental chamber (FIO2 = 14.5%) for >12 hours per day and the LLTL group at sea level under comfortable conditions. The hematological parameters showed no significant interaction. However, LHTL yielded more improved exercise economy, metabolic parameters (oxygen consumption=-152.7 vs 32.4 mL·kg-1·30min-1, η2 = 0.457, p = 0.000; tissue oxygenation index=6.18 vs .66%, η2 = 0.250, p = 0.013), and hemodynamic function (heart rate = -234.5 vs -49.7 beats·30min-1, η2 = 0.172, p = 0.044; stroke volume = 136.4 vs -120.5 mL/30 min, η2 = 0.191, p = 0.033) during 30 minutes of submaximal cycle ergometer exercise corresponding to 80% maximal heart rate before training than did LLTL. Regarding exercise performance, LHTL also yielded more improved VO2max (5.40 vs 2.35 mL·kg-1·min-1, η2 = 0.527, p = 0.000) and 3,000 m time trial performance (-34.0 vs -19.5 seconds, η2 = 0.527, p = 0.000) than did LLTL. These results indicate that compared with LLTL, LHTL can have favorable effects on exercise performance by improving exercise economy and hemodynamic function in competitive runners. |
Key words:
Living high-training low, erythropoietin, exercise economy, hemodynamic function, exercise performance, competitive runner
|
Key
Points
- Twenty-one days of LHTL involving more than 12 hours per day of residence at 3,000 m (F1O2=14.5%) in a normobaric hypoxic environment and more than 4 hours per day of training at sea level improves exercise performance compared with LLTL in moderately trained, competitive runners.
- The improvement in exercise performance (e.g., VO2max and 3,000 m time trial performance) observed after 21 days of LHTL might be attributed to the increase in exercise economy (e.g., VO2 and TOI) and hemodynamic function (e.g., HR and SV) during submaximal exercise compared with that in LLTL.
|
Exercise performance is highly related to various factors that can be altered through altitude/hypoxic training, including erythropoiesis, exercise economy (defined as the amount of energy spent per unit of distance), metabolic response, aerobic exercise capacity, and capillary density (Levine and Stray-Gunderson, 1997; Park et al., 2017; Saunders et al., 2004). Therefore, many sports scientists have been studying various altitude/hypoxic training programs aiming to enhance the exercise performance of athletes; currently, altitude/hypoxic training is recognized as a useful training method for athletes to improve their performance potentially in subsequent competitions at sea level (Brocherie et al., 2015; Millet et al., 2013; Sinex and Chapman, 2015). Altitude/hypoxic training aiming to improve athletic performance can be classified into four types: living high-training high (LHTH); living low-training high (LLTH), such as intermittent hypoxic exposure, continuous hypoxic training, interval hypoxic training (IHT), and repeated sprint training in hypoxia (RSH); living high-training low (LHTL); and living high-training low and high, such as LHTL + IHT or RSH (Brocherie et al., 2015; Millet et al., 2013; Wilber, 2007). To date, LHTL is widely recognized as a relatively effective altitude/hypoxic training method for improving athletic performance at sea level, despite ongoing debate (Bonetti and Hopkins, 2009; Brugniaux et al., 2006; Garvican et al., 2011). The potential underlying mechanisms for improved exercise performance following LHTL include increase in erythropoiesis and blood oxygen transporting capacity (Hauser et al., 2017; Levine and Stray-Gunderson, 1997; Wehrlin et al., 2006). This translates into increased maximal oxygen consumption (VO2max) while residing in an altitude/hypoxic environment, and there is a possibility that the increased exercise intensity in competitions and enhanced VO2max will be maintained at sea level (Levine, 2002; Schmitt et al., 2006; Wilber, 2007). LHTL improves the sea-level running performance of trained elite runners because of adaptation to hypoxia (increase in erythropoiesis and VO2max) and maintenance of training velocity at sea level, most likely accounting for the increase in running velocity at VO2max (Brugniaux et al., 2006; Garvican et al., 2011). These benefits are primarily attributed to the increase in erythropoiesis that occurs while residing in an altitude/hypoxic environment, provided that the “dose” of hypoxia is adequate (Dehnert et al., 2002; Levine and Stray-Gundersen, 2006; Wehrlin and Marti, 2006; Wehrlin et al., 2006). However, several studies have indicated that LHTL does not increase erythropoiesis or exercise performance (Ashenden et al., 1999a; Clark et al., 2004; Hahn et al., 2001). Ashenden et al. (1999a) demonstrated that 12 nights of exposure to moderate normobaric hypoxia (e.g., at an altitude of 2,650 m) is not sufficient to stimulate erythropoiesis. Hahn et al. (2001) reported that failure of moderate hypobaric hypoxia (e.g., at an altitude of 2,500 m) for 23 days to stimulate erythropoiesis and VO2max improvement is probably attributable to an insufficient duration of exposure (8–11 hours daily). In addition, Clark et al. (2004) reported that exercise economy, muscle markers of lactate metabolism, and pH regulation were unchanged after 20 consecutive nights of normobaric hypoxic exposure (fraction of inspired oxygen [FIO2], 16.27%). These previous studies have indicated that LHTL is affected not only by enhanced erythropoiesis but also by improved exercise economy, increased storage of glycogen, utilization of fatty acids, muscle buffer capacity, skeletal muscle oxygenation, and cardiovascular function (Garvican et al., 2011; Gore et al., 2001; Park and Nam, 2017; Saunders et al., 2004). Therefore, to assess the effectiveness of LHTL in improving athletic performance, various physiological mechanisms, such as exercise economy, muscle buffer capacity, skeletal muscle oxygenation, cardiovascular function, and erythropoiesis, must be considered. Another issue that has been raised regarding the effectiveness of LHTL in improving exercise performance is the optimal dose of hypoxia. Levine and Stray-Gundersen (2006) recommended using hypoxic environments at altitudes ranging from 2,000 to 3,000 m to stimulate erythropoiesis and to avoid any detrimental effects, such as acute mountain sickness, leukocyte count alteration, ventilator response impairment, and desaturation. Brugniaux et al. (2006) sought to determine the influence of factors, such as range and duration of residence in/exposure to the altitude/hypoxic environment, on the balance between the beneficial effects and potentially detrimental effects of LHTL. They suggested that during LHTL, the altitude should not exceed 3,000 m; residence should be continued for at least 18 days; and exposure should be at a minimum of 12 hours per day. Based on previous findings, we established the following hypothesis: 21 days of LHTL involving more than 12 hours per day of residence at 3,000 m (FIO2 = 14.5%) in a normobaric hypoxic environment and more than 4 hours per day of training at sea level will improve exercise performance by enhancing erythropoiesis, exercise economy and metabolism, and hemodynamic function during submaximal exercise compared with LLTL in moderately trained competitive runners.
ParticipantsThe participants were male, competitive, moderately trained, middle- and long-distance runners (n = 24) registered with the Korea Association of Athletics Federations. They were randomly assigned to perform either LLTL (n = 12) or LHTL (n = 12) according to their body composition, VO2max, and 3,000 m time trial performance (Table 1). All 12 participants in the LHTL group resided together in environmental chambers comprising a 14.5% oxygen normobaric hypoxic environment. The 12 participants in the LLTL group resided together in a room at sea level. Other than oxygen concentration, the remaining environmental conditions during residence were the same (temperature = 24 ± 2°C; humidity = 60 ± 2%) for the LHTL and LLTL groups. During the 21-day intervention period, the weekly routine for all athletes included 6 days of training and 1 day of rest. The participants received information on the study purpose and methods. They provided written consent for participation after receiving sufficient explanations regarding the experiment and understanding the possible adverse effects prior to the start of the study. This study was approved by our Institutional Review Board (7001355-201510-HR-090) and was conducted in accordance with the provisions of the Declaration of Helsinki.
Study designThe experimental design is shown in Figure 1 and consisted of the following: 7-day preintervention period (i.e., 3 testing days and 2 rest days between testing days) at sea level, during which baseline testing was performed; 21-day living and training session in the assigned environmental condition; and 7-day postintervention period at sea level. During the 3-day testing sessions, hematological parameters, exercise economy and metabolism, hemodynamic function, and exercise performance were evaluated. On the first testing day, hematological parameters, including erythrocyte count, hemoglobin (Hb) concentration, hematocrit (Hct), reticulocyte count, erythropoietin (EPO) concentration, mean corpuscular volume (MCV), mean corpuscular Hb (MCH), and MCH concentration (MCHC), were measured between 7:00 and 8:00 AM in the rested state. Thereafter, the body composition was measured. Subsequently, the VO2max was measured to evaluate exercise performance in the afternoon. On the second testing day, the following parameters were measured during a 30-minute bout of submaximal cycle ergometer exercise: exercise economy; metabolism indicators, including oxygen consumption (VO2) and blood lactate concentration; skeletal muscle oxygenation, including oxygenated Hb and myoglobin (O2Hb), deoxygenated Hb and myoglobin (HHb), and tissue oxygenation index (TOI); and hemodynamic function, including heart rate (HR), stroke volume (SV), end-diastolic volume (EDV), end-systolic volume (ESV), and cardiac output (CO). Exercise intensity was set at individual cycle ergometer exercise load values corresponding to 80% maximal HR (HRmax) obtained during the preintervention period. On the third testing day, performance in a 3,000 m time trial was measured. After the preintervention period, the participants were randomly assigned to one of two groups according to their initial body composition, VO2max, and 3,000 m time trial performance (Table 1): LHTL group (21-day LHTL, which included >12 hours per day of living in a normobaric hypoxic environment at an altitude of 3,000 m and training at sea level [n=12]) and LLTL group (21-day LLTL, which included living and training at sea level [n=12]). The athletes in the LHTL group stayed in a 10×14×3 m3 environmental chamber (Submersible Systems, Huntington Beach, CA, USA). The 3,000 m altitude (FIO2=14.5%) normobaric hypoxic environment was simulated by introducing nitrogen into the environmental chamber using a nitrogen generator (Separation & Filter Energy Technology Cooperation, Siheung, Korea). The athletes in the LLTL group resided at sea level under comfortable conditions otherwise similar to those for the LHTL group. The daily schedule of the 21-day program is shown in Table 2. The training program was created by coaches based on their experience and does not conform to regular practice. The daily training programs consisted of >4 hours of exercises, including interval rests performed at various intensities, which were expressed as percentages of HRmax. As shown in Table 3, the daybreak exercise consisted of a 20-minute warm-up at 45–50% HRmax, 60-minute jogging at 70–80% HRmax, and 20-minute cool-down at 45–50% HRmax. The morning exercise consisted of a 20-minute warm-up at 45–50% HRmax, six bouts of high-speed running over a distance of 150 m at 90–95% HRmax, four bouts of interval running over a distance of 1,200 m at 85–95% HRmax, and 20-minute cool-down at 45–50% HRmax. Finally, the afternoon exercise consisted of a 20-minute warm-up at 45–50% HRmax, five bouts of high-speed running over a distance of 300 m at 90–95% HRmax, 3,000 m or 5,000 m time trial, and 20-minute cool-down at 45–50% HRmax. During the study period, three training managers (a coach, trainer, and researcher) supervised all training courses. However, the subjects were not able to complete the exercise training at the prescribed intensity in our study for 21 days. All participants received oral liquid iron supplementation (FeoSol; 120 mg elemental iron/mL) with the dose adjusted on the basis of the plasma ferritin concentration (range, 5–45 mL·day-1).
MeasurementBody composition (i.e., height, weight, fat-free mass, and percent body fat) was evaluated after an overnight fast using bioelectrical impedance analysis, which measures the body’s resistance (impedance) to the flow of alternating electrical current (at a designated frequency) between two points of contact on the body. Because the water in the body tissues is conductive, the measurement of body impedance can indirectly provide information on the content of various tissues, including total body water, fat-free mass, and skeletal muscle mass (Talma et al., 2013). All participants wore lightweight clothing and were asked to remove any metal items. An Inbody 770 device (Inbody, Seoul, Korea) was used to measure body composition. To analyze hematological parameters, blood samples were obtained before and after intervention at rest at sea level. A 6-mL sample of venous blood was collected in a heparin tube for whole blood (5 mL) and a serum separation tube for serum (1 mL). The heparin tube for whole blood was inverted gently several times immediately after blood collection to ensure anti-coagulation, and the tube was stored in a refrigerator (-20°C) until the time of the assay. The blood sample in the serum separation tube was centrifuged at 3,500 rpm for 10 minutes. The serum obtained was also stored at -70°C until the time of the assay. To analyze the erythrocyte count, Hb concentration, Hct, and reticulocyte count, an XE2100D hematology analyzer (Sysmex, Kobe, Japan) was used. The erythrocyte count and Hct were measured using an impedance-based method; Hb concentration, cyanide-free Hb spectrophotometry; reticulocyte count, flow cytometry; and EPO concentration, Immulite 2000 XPI analyzer (Siemens, Eschborn, Germany) via the chemiluminescent immunoassay method. The MCV, MCH, and MCHC were calculated using the following formulas: MCV = (Hct/erythrocyte) × 10; MCH = (Hb/erythrocyte) × 10; and MCHC = (Hb/Hct) × 100. To evaluate exercise performance and intensity, the VO2max was measured before and after the intervention using the McArdle protocol (McArdle et al., 1973) for graded exercise testing on a cycle ergometer (Monark Exercise AB, Vansbro, Sweden) using a Vmax-229 breath-by-breath auto metabolism analyzer (SensorMedics, Yorba Linda, CA, USA). It starts at 900 kg·m·min-1 (~150 W) and increases by 180 kg·m·min-1 (~30 W) every 2 minutes until exhaustion. The pedal frequency was set at 60 rpm. The VO2max was the highest VO2 obtained during the concluding period of the exercise test. The graded exercise test was completed when the following three items were satisfied: 1) VO2 plateau - no further increase in oxygen use per minute even with an increase in work performed, 2) HR within 10 beats of the age-predicted HRmax - this is the basis for using participants’ HRmax as a surrogate for the VO2max when designing personal training programs, and 3) plasma (blood) lactate concentrations of >7 mmol/L. The 3,000 m time trial performance was measured on a 400-m track at sea level in Seoul between 9:00 and 10:00 AM (temperature=22–24°C; humidity=60–80%; wind=0–10 km/h). To avoid the effect of racing strategies, all starts were staggered by at least 2 minutes. Exercise economy, metabolism response, and hemodynamic function were measured before and after training while the participants performed submaximal cycle ergometer exercise for 30 minutes at sea level. The VO2 was measured using the Vmax-229 breath-by-breath auto metabolism analyzer and a breathing valve in the form of a facemask. To analyze the blood lactate concentration, blood (80 μL) was collected in a capillary tube using the fingertip method, and the sample was analyzed using the YSI-1500 lactate analyzer (YSI Inc., Yellow Springs, OH, USA). Skeletal muscle oxygenation profiles of the right vastus lateralis were evaluated using a commercially available near-infrared spectroscopy (NIRS) system (Hamamatsu NIRO-200; Hamamatsu Photonics, Hamamatsu, Japan) (Park et al., 2018). The intensities of incident and transmitted light were recorded continuously and used for online estimation of the changes from baseline (Δ%) of the concentrations of the O2Hb, HHb, and total Hb (HbTOT=Hb+myoglobin) along with the relevant specific extinction coefficients. TOI values were calculated using the following formula: 100×O2Hb/HbTOT. The HR, SV, EDV, ESV, and CO were assessed noninvasively using a thoracic bioelectrical impedance device (PhysioFlow PF-05, Paris, France). All variables were measured every minute, except for the blood lactate concentration, which was measured at 10, 20, and 30 minutes into the exercise. In the subsequent analysis, the total value was used for the VO2, HR, SV, EDV, ESV, and CO, while the average value was used for the O2Hb, HHb, TOI, and blood lactate concentration.
Statistical analysisAll statistical analyses were conducted using the SPSS software version 23.0 (IBM Corp., Armonk, NY, USA) for Windows. Data were presented as means ± standard deviations. To determine the sample size, we focused on identifying meaningful differences in the VO2 during submaximal exercise, as previously suggested (Park et al., 2017). The a priori power analysis, which was performed using G-power, indicated that a minimum sample size of 20 participants (10 participants per group) is required to provide 80% power at an α level of 0.05. Anticipating a dropout rate of >10%, we aimed for a starting sample size of 24 participants. Normality of distribution of all outcome variables was verified using the Kolmogorov-Smirnov test. A two-way analysis (time × group) of variance with repeated measures of the “time” factor was used to analyze the effects of the training programs on each dependent variable. If a significant interaction effect was found, the Bonferroni post-hoc test was used to identify within-group changes over time. Additionally, the paired t-test was used to compare between the post-training and pre-training values of the dependent variables in each group separately. The level of significance was set a priori at 0.05.
Hematological parametersAs shown in Table 4, there was no significant interaction regarding all hematological parameters. The 21-day LHTL performed in our study did not affect the hematological parameters, such as erythrocyte count, Hb concentration, Hct, reticulocyte count, EPO concentration, MCV, MCH, and MCHC, compared with LLTL in the competitive runners.
Exercise economy and metabolismNo significant interaction was observed regarding the O2Hb, HHb, and blood lactate concentration; however, the VO2 (η2 = 0.457, p < 0.001) and TOI (η2 = 0.250, p = 0.013) showed significant interactions. After the intervention, the post-hoc analyses indicated that the LHTL group showed a significant decrease in VO2 (mean change = -152.7; 95% confidence interval [CI] = -237.2, -68.1; p < 0.05) and a significant increase in TOI (mean change = 6.18; 95% CI=3.14, 9.21; p < 0.05) during the 30-minute bout of submaximal cycle ergometer exercise corresponding to 80% HRmax obtained during the preintervention period (Table 4).
Hemodynamic functionThere was a significant interaction for the HR (η2 = 0.172, p = 0.044) and SV (η2 = 0.191, p = 0.033). Compared with the LLTL group, the LHTL group showed a significant decrease in HR (mean change = -234.5; 95% CI = -346.7, -122.3; p < 0.05) and a significant increase in SV (mean change = 136.4; 95% CI = 22.6, 295.5; p < 0.05) during the 30-minute bout of submaximal cycle ergometer exercise corresponding to 80% HRmax obtained during the preintervention period. However, no significant interaction was observed regarding the EDV, ESV, or CO (Table 4).
Exercise performanceThere was a significant interaction for the VO2max (η2 = 0.527, p < 0.001) and 3,000 m time trial performance (η2 = 0.217, p = 0.022). The post-hoc analyses found significant improvements in both groups for the VO2max (LHTL: mean change = 5.40; 95% CI = 4.32, 6.48; p < 0.05, LLTL: mean change = 2.35; 95% CI = 1.54, 3.17; p < 0.05) and 3,000 m time trial performance (LHTL: mean change=-34.0; 95% CI = -45.6, -22.4; p < 0.05, LLTL: mean change = -19.5; 95% CI = -25.1, -13.8; p < 0.05). However, the LHTL group showed a greater improvement in exercise performance than did the LLTL group (Table 4).
Hematological parametersIf an effective hypoxia dose (appropriate exposure level, exposure time, and exposure duration in hypoxia) is provided, exposure to altitude/hypoxic environments enhances erythropoiesis, thus leading to increased VO2max and exercise performance (Dehnert et al., 2002; Levine and Stray-Gundersen, 2006; Robach et al., 2006; Schmitt et al., 2006). Levine and Stray-Gunderson (1997) reported that 4 weeks of LHTL improved the sea-level running performance of trained runners owing to increased red cell mass, red cell volume, and VO2max compared to that with LLTL. Robach et al. (2006) demonstrated that 13 days of LHTL at simulated altitudes of 2,500 m and 3,000 m was sufficient to elicit a substantial increase in red blood cell volume in highly trained swimmers; however, a clear improvement in aerobic performance was not reported. Wehrlin et al. (2006) concluded that living at 2,500 m and training at a lower altitude for 24 days increased Hb mass (Hbmass) and red cell volume compared with LLTL. Conversely, Hahn et al. (2001) analyzed six previous studies and concluded that living in a hypoxic environment at 2,650–3,000 m for up to 23 days may offer practical benefits to elite athletes; however, any observed effect is not likely attributed to increased Hbmass or VO2max. Other previous studies also reported no changes in reticulocyte volume or Hbmass after 12 nights at 2,650 m (Ashenden et al., 1999a), 23 nights at 3,000 m (Ashenden et al., 1999b), or 14 nights at 1,956 m (Dehnert et al., 2002). Although we did not measure the red cell volume and red blood cell mass in this study, 21 days of LHTL did not affect the hematological parameters compared with LLTL. Our finding is unique in this regard because other researchers have reported mean increases of 5–9% in red cell volume and >8% in Hb concentration (Hahn et al., 2001; Levine and Stray-Gunderson, 1997; Rusko et al., 1999; Stray-Gunderson et al., 2001). It is important to note that we found no difference in the hematological parameters between LHTL and LLTL, despite the fact that the participants in the present study received oral liquid iron supplementation with the dose adjusted on the basis of the plasma ferritin concentration (120 mg elemental iron/mL/day). We consider that the reason for the absence of changes in the hematological parameters via LHTL is that the red cell volume and red blood cell mass, which represent erythropoiesis, were not measured. Therefore, our 21 days of LHTL involving more than 12 hours per day of living in a 14.5% oxygen hypoxic environment and 4 hours per day of training at sea level did not improve the hematological parameters.
Exercise economy and metabolismThe VO2max and exercise economy are widely known as determinants of exercise performance (velocity in human locomotion) (Rusko et al., 2004; Schmitt et al., 2006). In particular, exercise economy is highly correlated with exercise performance (Saunders et al., 2004) and is recognized as an accurate predictive factor of exercise performance (Gore et al., 2001; Green et al., 2000). The following mechanisms may underlie the increase in exercise economy: an increase in the amount of ATP produced per mole of oxygen and a decrease in the amount of ATP used during submaximal exercise at the same intensity (speed or load). Although they did not include a control group with the same physical activity at sea level, Green et al. (2000) reported a significant improvement in the net mechanical economy of submaximal cycling after a 21-day mountaineering expedition at 6,194 m. Gore et al. (2001) reported that in male athletes, LHTL increased muscle buffer capacity and exercise economy during exercise after intervention involving sleeping for 23 nights at a moderate simulated altitude (3,000 m) and training near sea level (600 m). Katayama et al. (2003) also reported that both prolonged and intermittent hypoxic exposures increased exercise economy during submaximal exercise at sea level among 12 elite runners. Recently, Park et al. (2017) suggested that 4 weeks of LHTL effectively enhances 3,000 m and 5,000 m time trial performance by achieving enhanced exercise economy and metabolism (HR, VO2, VCO2, and blood lactate concentration) during submaximal exercise. LHTL may increase exercise economy via two possible underlying mechanisms (Levine, 2002). First, acclimation to altitude/hypoxic environments causes increased use of carbohydrates with oxidative phosphorylation. Chronic exposure to altitude/hypoxic environments for 20 days was related to free fatty acid uptake decreases, whereas carbohydrate metabolism increased during 50% VO2max cycle ergometer exercise in 11 nonsmoking, untrained men living at sea level (Roberts et al., 1996; Saunders et al., 2004). Second, metabolic adaptation to an altitude/hypoxic environment may increase the muscular hemodynamic function (Green et al., 2000; Park et al., 2017). To confirm the second mechanism, we measured skeletal muscle oxygenation profiles of the right vastus lateralis during a 30-minute bout of submaximal cycle ergometer exercise using a commercially available NIRS system (Kawaguchi et al., 2006; Park and Nam, 2017). The NIRS signal reflects the dynamic balance between muscle capillary blood flow and muscular VO2 in the microcirculation (Wang et al., 2014). In a study of the changes in skeletal muscle oxygenation profiles (ΔO2Hb, ΔHHb, and TOI) after LHTL, Park and Nam (2017) reported that LHTL (living at a simulated altitude of 3,000 m and training at an altitude of 700–1,330 m) did not significantly affect the ΔO2Hb, ΔHHb, or TOI in middle- and long-distance college runners. However, our study revealed that the TOI (100×O2Hb/HbTOT) enhancement was significantly more pronounced after LHTL than after LLTL. Taken together, these findings suggest that LHTL improves the dynamic balance between muscle capillary blood flow and muscular VO2 in the microcirculation, potentially improving oxygen delivery and utilization capacity in the muscle. Thus, we confirmed that the 21-day LHTL regimen involving more than 12 hours per day of living in a 14.5% oxygen hypoxic environment and 4 hours per day of training at sea level has a positive effect on exercise economy (VO2 during submaximal exercise) and metabolism (dynamic balance between muscle capillary blood flow and muscular VO2 in the microcirculation).
Hemodynamic functionPrevious studies have reported that LHTL involving residence in a hypoxic environment and intense training at sea level increases exercise economy and performance by improving oxygen transport capacity in the blood and tissues (Brocherie et al., 2015; Katayama et al., 2003; Park et al., 2017). Among the physiological factors that affect exercise performance, the oxygen delivery capacity of the blood has an important role in hemodynamic function (Park and Nam, 2017). Liu et al. (1998) reported that LHTL increases energy availability through the stimulation of the parasympathetic nervous system, which is reflected in the activation of β-adrenergic receptors in the cardiac muscles and results in improved SV with enhanced left ventricular contractility. Reeves et al. (1992) showed that adaptation to a hypoxic environment leads to an increase in blood pressure and vascular tone via α-adrenergic receptor stimulation in the cardiac muscle and an increase in norepinephrine concentrations in the blood and urine. Park and Nam (2017) reported that compared with LLTL, LHTL can yield favorable effects for cardiac function by improving the HR, SV, EDV, and ESV during submaximal exercise in athletes. Our study revealed that compared with LLTL, LHTL induced a significant decrease in HR and increase in SV during submaximal exercise. However, no significant changes in the EDV or ESV were noted, despite the substantial effect of LHTL on the SV. These findings may be partially explained by the greater tendency to increase in the ESV noted after LLTL than after LHTL (36.7% vs 13.9%), although the EDV showed a similar tendency to increase in the two groups (LLTH vs LLTL, 7% vs 9.8%). Therefore, the more substantial increase in the SV noted after LHTL is likely related mainly to differences in the ESV. Our results are consistent with those of several studies that showed that improvements in ventricular contractility through the ESV and SV should result from activation of β-adrenergic receptors and increased energy efficiency in the cardiac muscles (Li et al., 1996; Liu et al., 1998). Furthermore, the decrease in the HR noted in the LHTL group might be related to decreased sympathetic nerve activity and increased parasympathetic nerve activity, resulting in activation of α2-adrenergic receptors. However, our data do not provide a basis to infer sympathetic or parasympathetic activity. Therefore, it is difficult to conclude that the LHTL-induced decrease in the HR and increase in the SV during submaximal exercise are attributed to the activity of the parasympathetic nervous system (via α2-adrenergic receptors) and sympathetic nervous system (via β-adrenergic receptors), as discussed in previous studies. Moreover, our data indicated that after LHTL, the CO did not improve with the HR and SV (Liu et al., 1998; Park and Nam, 2017).
Exercise performanceSeveral studies have indicated that the efficacy of LHTL in improving the exercise performance of collegiate and elite runners is based on enhancing oxygen transport ability (Brugniaux et al., 2006; Garvican et al., 2011; Robach et al., 2006; Sinex and Chapman, 2015). Most previous studies have suggested that enhanced oxygen transporting ability (e.g., erythrocyte volume and Hbmass) may affect exercise performance (VO2max and time trial performance). Levine and Stray-Gunderson (1997) indicated that 4 weeks of LHTL increases sea-level running performance in trained runners by enhancing erythropoiesis (red cell mass) and aerobic exercise capacity (VO2max and velocity at VO2max). Regarding the efficacy of LHTL compared with LHTH, LHTL has a positive effect on exercise performance by retaining training speed and oxygen flux during high-intensity interval training; however, LHTH lowers the VO2, HR, and peak lactate concentration during exercise with established intensity. Stray-Gunderson et al. (2001) studied 22 elite runners before and after 27 days of living at 2,500 m while performing high-intensity training at 1,250 m and concluded that LHTL improves exercise performance and maximal oxygen transport capacity even for elite runners; the improvement manifested in terms of endurance performance (3%), EPO concentration (×2), 3,000 m time trial performance (1.1%), and personal best records (1/3 subjects). Wehrlin et al. (2006) concluded that living at 2,500 m and training at 500–1,600 m for 24 days increase the Hbmass and red cell volume in elite orienteers and that such changes may enhance performance in elite endurance athletes. Christoulas et al. (2011) reported that living at 1,550–2,050 m and training at 450–500 m for 21 days significantly increase the red cell mass and VO2max in cross-country skiers. Our study showed that the LHTL group had significantly more pronounced increases in the VO2max and 3,000 m time trial performance than the LLTL group, whereas no hematological parameters showed improvements attributable to LHTL; importantly, we could not conclude that LHTL improves oxygen transport capacity. In contrast with the favorable effects on exercise performance (as reported in previous studies), exercise performance may require improvement in the oxygen delivery capacity that relies not only on hematological parameters but also on other factors. Robach et al. (2006) reported that LHTL (training at 1,200 m while residing at 2,500 or 3,000 m) for 13 days had a positive effect on the red blood cell volume in swimmers; however, this hematological response was not associated with a clear concomitant improvement in aerobic performance. Similarly, Robertson et al. (2010) concluded that 21 days of LHTL (training below 500 m while residing at 3,000 m) can induce a reproducible mean improvement in the Hbmass and VO2max in elite athletes; however, this enhanced physiological capacity did not translate in improved 4–5-km time trial performance. Our results indicate that the improvement in exercise performance (e.g., VO2max and 3,000 m time trial performance) observed after 21 days of LHTL might be attributed to the increase in exercise economy (e.g., VO2 and TOI) and hemodynamic function (e.g., HR and SV) during submaximal exercise.
Limitations
- Our study has some limitations in its methodology. We measured the VO2max and HRmax using the graded exercise test and exercise economy, metabolism, and hemodynamic function during submaximal exercise using a bicycle in middle- and long-distance competitive moderately trained runners. First, when measuring cardiac function and skeletal muscle oxygenation using the PhysioFlow PF-05 Lab1 and Hamamatsu NIRO-200 during exercise on a treadmill, the reliability of the data is reduced owing to the severe noise. Therefore, we employed the graded exercise test using a treadmill. The VO2max test can be further performed using a treadmill; however, this puts a heavy burden on the athletes, and we have to consider the match schedule before and after the study. Second, we attempted to measure the exercise economy, metabolism, and hemodynamic function during 30 minutes of submaximal bicycle exercise with exercise load values corresponding to 80% VO2max obtained at preintervention. To measure the exercise intensity for submaximal bicycle exercise, we performed the graded exercise test on a bicycle to determine the VO2max. The submaximal exercise test was performed with the same bicycle load (corresponding to 80% VO2max obtained at preintervention) before and after training. Further, we have not identified a previous study that used a graded bicycle exercise test to measure competitive runners’ exercise performance. Therefore, not measuring the VO2max and HRmax in a treadmill is an obvious limitation of this study.
- According to Chapman et al. (1998), to examine the changes in the EPO concentration by hypoxia, more time points (ideally baseline, 24 hours, 1 week, 2 weeks, and 21 days) must be considered. However, we conducted the analysis only before and after 21 days of intervention.
- We could not verify whether the prescribed training volume and intensity in all subjects were achieved.
- Despite the reported physiological differences between prolonged exposure to normobaric hypoxia (simulated altitude) and hypobaric hypoxia (simulated and terrestrial altitudes) (Debevec et al., 2015; Saugy et al., 2015), a normobaric hypoxic condition for living high was applied to the competitive runners. Compared with normobaric hypoxia, hypobaric hypoxia is known to induce a higher improvement in endurance performance by achieving greater oxidative stress and hypoxic stress at rest and during exercise.
- Neither the athletes nor the experimenters were blinded to the intervention.
Our study demonstrated that 21 days of LHTL is very effective in enhancing exercise performance (VO2max and 3,000 m time trial performance) through improved exercise economy and hemodynamic function compared to that with LLTL in moderately trained, competitive, middle- and long-distance runners.
ACKNOWLEDGEMENTS |
This study was supported by a grant (NRF-2015M3C1B1019479) from the National Research Foundation funded by the Korean Government. The experiments comply with the current laws of the country in which they were performed. The authors have no conflict of interest to declare. |
|
AUTHOR BIOGRAPHY |
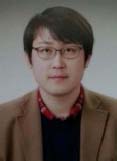 |
Hun-Young Park |
Employment: Assistant Professor (Sports Science) at Physical Activity and Performance Institute |
Degree: PhD |
Research interests: Training effect in hypoxic condition for sports performance, Clinical effects related to obesity and cardiovascular disease by exposure or training in hypoxic condition. |
E-mail: parkhy1980@konkuk.ac.kr |
|
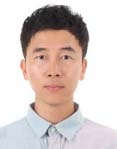 |
Wonil Park |
Employment: Doctoral Student at Korea University |
Degree: MS |
Research interests: Responses of vascular system at rest, during exercise, and after exercise in individuals with chronic conditions. |
E-mail: wonilpark01@korea.ac.kr |
|
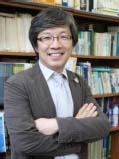 |
Kiwon Lim |
Employment: Professor of Exercise Nutrition (Department of Physical Education, Konkuk University, Korea) and Director at Physical Activity and Performance Institute |
Degree: PhD |
Research interests: Effect of long-term high fat diet and/or exercise on energy metabolism at rest and during exercise. Clinical effects related to obesity and cardiovascular disease by exposure or training in hypoxic condition. |
E-mail: exercise@konkuk.ac.kr |
|
|
|
REFERENCES |
 Ashenden M. J., Gore C. J., Dobson G. P., Hahn A. G. (1999b) “Live high, train low” does not change the total haemoglobin mass of male endurance athletes sleeping at a simulated altitude of 3000 m for 23 nights. European Journal of Applied Physiology and Occupational physiology 80, 479-484.
|
 Ashenden M. J., Gore C. J., Martin D. T., Dobson G. P., Hahn A. G. (1999a) Effects of a 12-day “live high, train low” camp on reticulocyte production and haemoglobin mass in elite female road cyclists. European Journal of Applied Physiology and Occupational Physiology 80, 472-478.
|
 Bonetti D. L., Hopkins W. G. (2009) Sea-level exercise performance following adaptation to hypoxia: a meta-analysis. Sports Medicine 39, 107-127.
|
 Brocherie F., Millet G. P., Hauser A., Steiner T., Rysman J., Wehrlin J. P., Girard O. (2015) Live high-train low and high” hypoxic training improves team-sport performance. Medicine & Science in Sports & Exercise 47, 2140-2149.
|
 Brugniaux J. V., Schmitt L., Robach P., Jeanvoine H., Zimmermann H., Nicolet G., Duvallet A., Fouillot J. P., Richalet J. P. (2006) Living high-training low: tolerance and acclimatization in elite endurance athletes. European Journal of Applied Physiology 96, 66-77.
|
 Chapman R. F., Stray-Gundersen J., Levine B. D. (1998) Individual variation in response to altitude training. Journal of Applied Physiology 85, 1448-1456.
|
 Christoulas K., Karamouzis M., Mandroukas K. (2011) “Living high - training low” vs. “living high - training high”: erythropoietic responses and performance of adolescent cross-country skiers. The Journal of Sports Medicine & Physical Fitness 51, 74-81.
|
 Clark S. A., Aughey R. J., Gore C. J., Hahn A. G., Townsend N. E., Kinsman T. A., Chow C. M., McKenna M. J., Hawley J. A. (2004) Effects of live high, train low hypoxic exposure on lactate metabolism in trained humans. Journal of Applied Physiology 96, 517-525.
|
 Debevec T., Pialoux V., Saugy J., Schmitt L., Cejuela R., Mury P., Ehrström S., Faiss R., Millet G. P. (2015) Prooxidant/Antioxidant Balance in Hypoxia: A Cross-Over Study on Normobaric vs. Hypobaric “Live High-Train Low”. PLoS One 10, e0137957-.
|
 Dehnert C., Hütler M., Liu Y., Menold E., Netzer C., Schick R., Kubanek B., Lehmann M., Böning D., Steinacker J. M. (2002) Erythropoiesis and performance after two weeks of living high and training low in well trained triathletes. International Journal of Sports Medicine 23, 561-566.
|
 Garvican L. A., Pottgiesser T., Martin D. T., Schumacher Y. O., Barras M., Gore C. J. (2011) The contribution of haemoglobin mass to increases in cycling performance induced by simulated LHTL. European Journal of Applied Physiology 111, 1089-1101.
|
 Gore C. J., Hahn A. G., Aughey R. J., Martin D. T., Ashenden M. J., Clark S. A., Garnham A. P., Roberts A. D., Slater G. J., McKennar M. J. (2001) Live high:train low increases muscle buffer capacity and submaximal cycling efficiency. Acta Physiologica Scandinavica 173, 275-286.
|
 Green H. J., Roy B., Grant S., Hughson R., Burnett M., Otto C., Pipe A., McKenzie D., Johnson M. (2000) Increases in submaximal cycling efficiency mediated by altitude acclimatization. Journal of Applied Physiology 89, 1189-1197.
|
 Hahn A. G., Gore C. J., Martin D. T., Ashenden M. J., Roberts A. D., Logan P. A. (2001) An evaluation of the concept of living at moderate altitude and training at sea level. Comparative Bio-chemistry and Physiology Part A: Molecular & Integrative Physiology 128, 777-789.
|
 Hauser A., Troesch S., Saugy J. J., Schmitt L., Cejuela-Anta R., Faiss R., Steiner T., Robinson N., Millet G. P., Wehrlin J. P. (2017) Individual hemoglobin mass response to normobaric and hypobaric “live high-train low”: a one-year crossover study. Journal of Applied Physiology 123, 387-393.
|
 Katayama K., Matsuo H., Ishida K., Mori S., Miyamura M. (2003) Intermittent hypoxia improves endurance performance and submaximal exercise efficiency. High Altitude Medicine & Biology 4, 291-304.
|
 Kawaguchi K., Hayashi Y., Sekikawa K., Tabusadani M., Inamizu T., Onari K., Bhambhani Y. (2006) Vastus lateralis oxygenation during prolonged cycling in healthy males. Applied Physiology, Nutrition, & Metabolism 31, 48-55.
|
 Levine B. D. (2002) Intermittent hypoxic training: fact and fancy. High Altitude Medicine & Biology 3, 177-193.
|
 Levine B. D., Stray-Gundersen J. (2006) Dose-response of altitude training: how much altitude is enough?. Advances Experimental Medicine & Biology 588, 233-247.
|
 Levine B. D., Stray-Gunderson J. (1997) “Living high-training low”: Effect of moderate-altitude exposure simulated with nitrogen tents. Journal of Applied Physiology 83, 102-112.
|
 Li H. T., Honbo N. Y., Karliner J. S. (1996) Chronic hypoxia increases β1-adrenergic receptor mRNA and density but not signaling in neonatal rat cardiac myocytes. Circulation 94, 3303-3310.
|
 Liu Y., Steinacker J. M., Dehnert C., Menold E., Baur S., Lormes W., Lehmann (1998) Effect of “living high-training low” on the cardiac functions at sea level. International Journal of Sports Medicine 19, 380-384.
|
 McArdle W. D., Katch F. I., Pechar G. S. (1973) Comparison of continuous and discontinuous treadmill and bicycle tests for VOmax. Medicine and Science in Sports 5, 156-160.
|
 Millet G. P., Faiss R., Brocherie F., Girard O. (2013) Hypoxic training and team sports: a challenge to traditional methods?. British Journal of Sports Medicine 47, i6-i7.
|
 Park H. Y., Kim S., Nam S. S. (2017) Four-week “living high training low” program enhances 3000 m and 5000 m time trials by improving energy metabolism during submaximal exercise in athletes. Journal of Exercise Nutrition & Biochemistry 21, 1-6.
|
 Park H. Y., Nam S. S. (2017) Application of “living high-training low” enhances cardiac function and skeletal muscle oxygenation during submaximal exercises in athletes. Journal of Exercise Nutrition & Biochemistry 21, 13-20.
|
 Park H. Y., Shin C., Lim K. (2018) Intermittent hypoxic training for 6 weeks in 3000 m hypobaric hypoxia conditions enhances exercise economy and aerobic exercise performance in moderately trained swimmers. Biology of Sport 35, 49-56.
|
 Reeves J. T., Mazzeo R. S., Wolfel E. E., Young A. J. (1992) Increased arterial pressure after acclimatization to 4300 m: possible role of norepinephrine. International Journal of Sports Medicine 13, S18-S21.
|
 Robach P., Schmitt L., Brugniaux J. V., Roels B., Millet G., Hellard P., Nicolet G., Duvallet A., Fouillot J. P., Moutereau S., Lasne F., Pialoux V., Olsen N. V., Richalet J. P. (2006) Living high–training low: effect on erythropoiesis and aerobic performance in highly-trained swimmers. European Journal of Applied Physiology 96, 423-433.
|
 Roberts A. C., Butterfield G. E., Cymerman A., Reeves J. T., Wolfel E. E., Brooks G. A. (1996) Acclimatization to 4,300 m altitude decreases reliance on fat as a substrate. Journal of Applied Physiology 81, 1762-1771.
|
 Robertson E. Y., Saunders P. U., Pyne D. B., Aughey R. J., Anson J. M., Gore C. J. (2010) Reproducibility of performance changes to simulated live high/train low altitude. Medicine & Science in Sports & Exercise 42, 394-401.
|
 Rusko H. K., Tikkanen H., Paavolainen L., Hämäläinen I., Kalliokoski K., Puranen A. (1999) Effect of living in hypoxia and training in normoxia on sea level VOmax and red cell mass. Medicine & Science in Sports & Exercise 31, S86.
|
 Rusko H., Tikkanen H., Peltonen J. (2004) Altitude and endurance training. Journal of Sports Sciences 22, 928-945.
|
 Saugy J. J., Schmitt L., Cejuela R., Faiss R., Hauser A., Wehrlin J. P., Rudaz B., Delessert A., Robinson N., Millet G. P. (2015) Correction: Comparison of “Live High-Train Low” in Normobaric versus Hypobaric Hypoxia. PLoS One 10, e0133091.
|
 Saunders P. U., Telford R. D., Pyne D. B., Cunningham R. B., Gore C. J., Hahn A. G., Hawley J. A. (2004) Improved running economy in elite runners after 20 days of simulated moderate-altitude exposure. Journal of Applied Physiology 96, 931-937.
|
 Schmitt L., Millet G., Robach P., Nicolet G., Brugniaux J. V., Fouillot J. P., Richalet J. P. (2006) Influence of “living high–training low” on aerobic performance and economy of work in elite athletes. European Journal of Applied Physiology 97, 627-636.
|
 Sinex J. A., Chapman R. F. (2015) Hypoxic training methods for improving endurance exercise performance. Journal of Sport & Health Science 4, 325-332.
|
 Stray-Gundersen J., Chapman R. F., Levine B. D. (2001) “Living high-training low” altitude training improves sea level performance in male and female elite runners. Journal of Applied Physiology 91, 1113-1120.
|
 Talma H., Chinapaw M. J., Bakker B., HiraSing R. A., Terwee C. B., Altenburg T. M. (2013) Bioelectrical impedance analysis to estimate body composition in children and adolescents: a systematic review and evidence appraisal of validity, responsiveness, reliability and measurement error. Obesity Review 14, 895-905.
|
 Wang J. S., Lee M. T., Lien H. Y., Weng T. P. (2014) Hypoxic exercise training improves cardiac/muscular hemodynamics and is associated with modulated circulating progenitor cells in sedentary men. International Journal of Cardiology 170, 315-323.
|
 Wehrlin J. P., Marti B. (2006) Live high-train low associated with increased haemoglobin mass as preparation for the 2003 World Championships in two native European world class runners. British Journal of Sports Medicine 40, e3.
|
 Wehrlin J. P., Zuest P., Hallén J., Marti B. (2006) Live high-train low for 24 days increases hemoglobin mass and red cell volume in elite endurance athletes. Journal of Applied Physiology 100, 1938-1945.
|
 Wilber R. L. (2007) Application of altitude/hypoxic training by elite athletes. Medicine & Science in Sports & Exercise 39, 1610-1624.
|
|
|
|
|
|
|